High-Speed Flight
Prime Movers Lab
February 2022
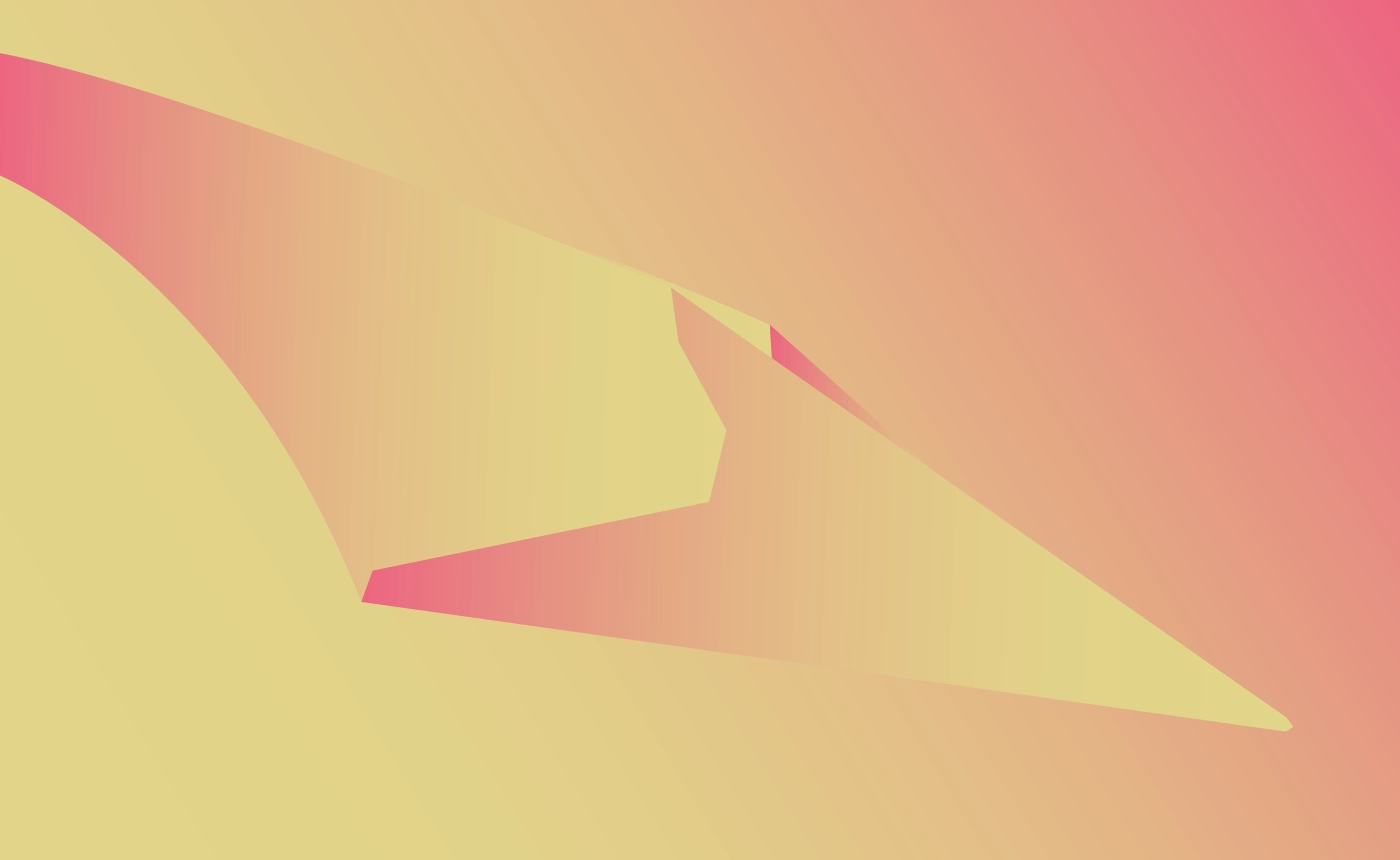
Over the last decade, I have been delighted to see the end of the ‘Great Stagnation’ in the aerospace industry. The upswing in aerospace technology development has been driven by a combination of advancements in materials and computers, the public’s increased appetite for technical risk, and the rise of a new Great Power Competition. This thought paper will review the fundamental physics, history, enabling technologies and current market landscape underpinning today’s high-speed flight renaissance.
Flow Physics
Before we dive into the current state-of-the-art, a definition of the basic terms and concepts of high-speed flow physics is warranted.
Supersonic flight denotes a vehicle whose speed (magnitude of the velocity vector) is faster than the local speed of sound (a). Aerodynamicists LOVE to non-dimensionalize so that it’s easier to compare their performance. For supersonic flight, the non-dimensional number of choice is the Mach number.
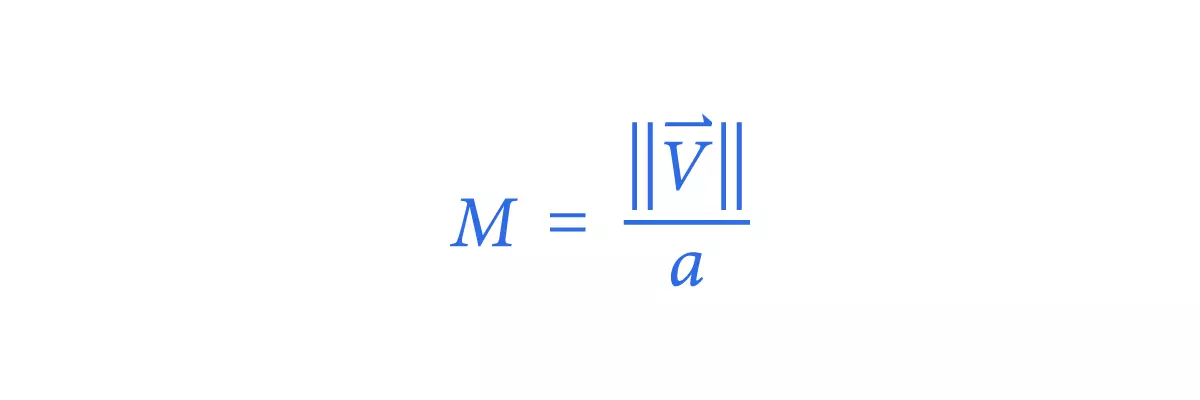
A Mach number greater than one (M>1) means you are in the supersonic flow regime. It’s worth noting that the speed of sound for an ideal gas is a function of the temperature. For an aircraft, you can think about temperature varying as a function of altitude, as measured in the 1976 Standard Atmosphere. Earth’s atmospheric temperature profile also varies both spatially and seasonally. This natural atmospheric variance is one of the main reasons why aircraft fuel performance varies depending on the route and its conditions.
How does supersonic flow differ from subsonic flow? Traveling faster than the speed of sound creates a local discontinuity in the flow, also known as a shock wave. There is an almost instantaneous step-change increase in static pressure, temperature, and density across the shock. At the same time the post-shock velocity shows a similar step-change decrease in value.
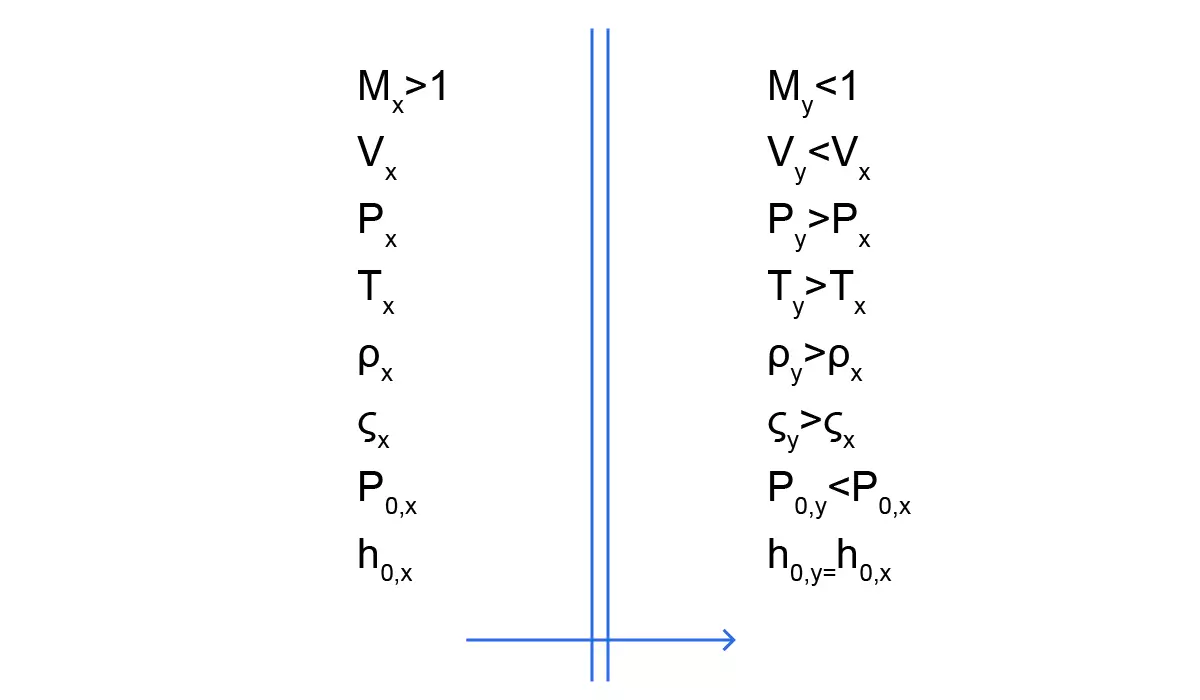
Change in flow properties across a normal shock
Flow physics in the supersonic flight regime are reasonably well modeled in one-dimension (1-D) via the normal shock relations. While the shock wave itself is an irreversible process, on either side of the shock the simplifying assumptions of isentropic flow (ideal gas, adiabatic, inviscid, irrotational flow) can be applied to get a closed-form solution. Rather than show equations, it’s helpful to view the normal shock relations plotted, to see how much each quantity changes with an increasing freestream Mach number.
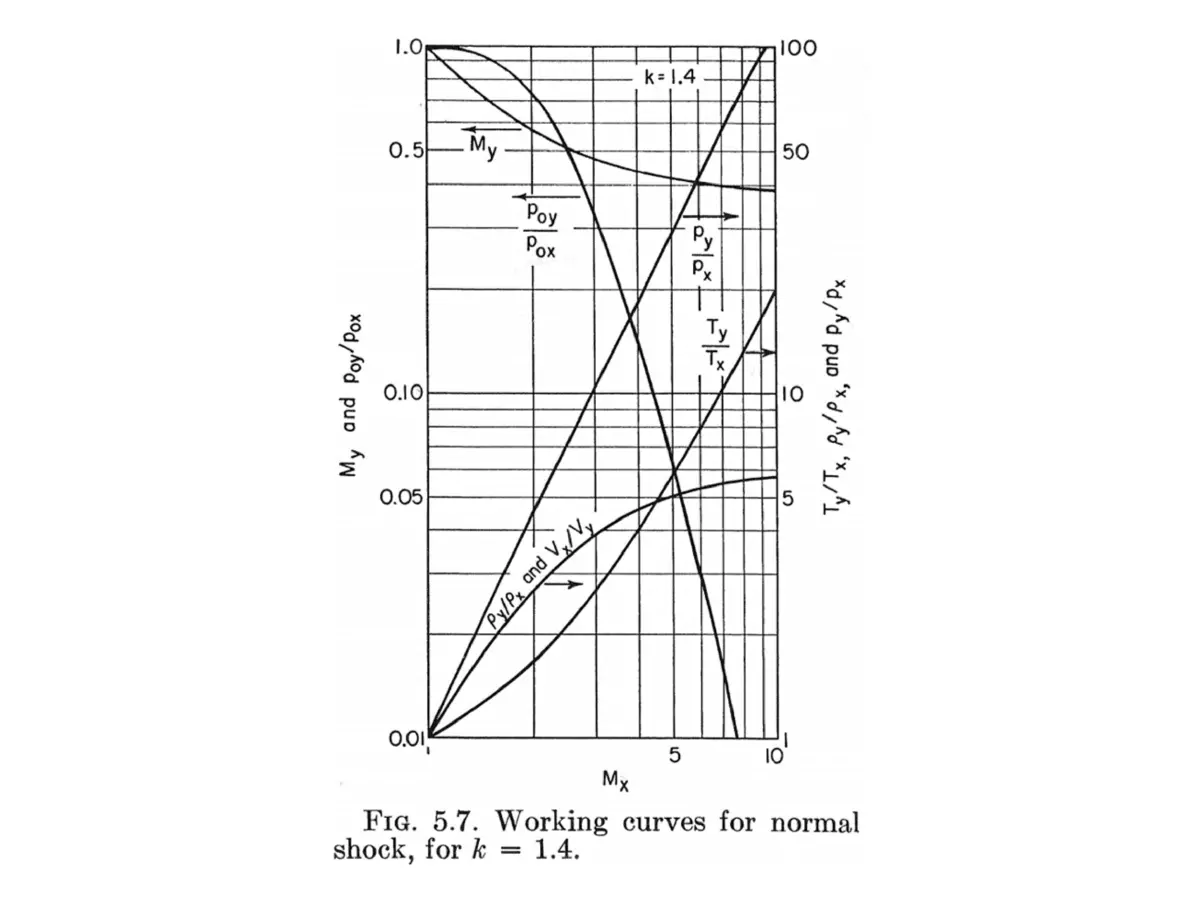
The normal shock relations for air[1]
You might ask, what’s the point of a 1-D model? Aircraft are 3-D! Aerodynamicists, in addition to loving non-dimensional numbers, also love scaling laws. They provide a framework for thinking about the largest first-order effects of the flow physics. In the diagram above, the biggest takeaway is that pressure scales with velocity squared.
The practical design implication for aircraft is that the faster you go, the larger the pressure loads and the more thrust you’ll need to overcome the resultant induced drag force. Pressure scales linearly with freestream density and linearly with the cross-sectional area of the aircraft, so aircraft designers have a few variables to trade during conceptual design. (Fun fact: subsonic aircraft cruise at a much lower altitude (~35,000 ft) than supersonic ones (~60,000ft). This is a design choice!)
Going from subsonic to supersonic flight has an immediate noticeable step-change in the flow physics caused by the shock wave. Conversely, when going from supersonic (Mach>1) to hypersonic (Mach>5) speeds, there is a more gradual breakdown of the simplifying assumptions for idealized flow. In the normal shock relations, the post-shock static temperature scales with Mach number cubed. For a freestream temperature of 245 K (atmospheric temperature at an altitude of 60km), the ideal gas post-shock temperature increases from ~400K at Mach 2, to ~1,400K at Mach 5, to ~12,000 K at Mach 15. As illustrated in the computational image below, hypersonic flow is non-ideal, with energy dissipation pathways that reduce the near-wall gas temperature closer to ~6,000 K. (The hypersonic flight environment is dominated by tremendous heat loads. This is still hotter than any existing material melting point. Materials will be covered in more detail later.)
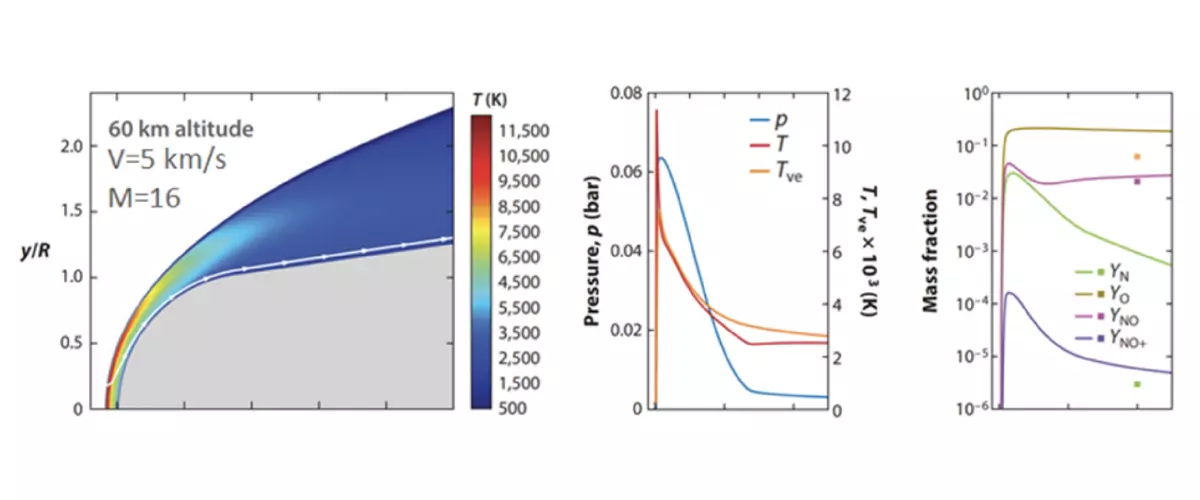
Computational Fluid Dynamics models illustrate thermochemical non-equilibrium of hypersonic flow. The blunt nose shape on the left pushes the highest temperature region away from the surface.[2]
As speed increases above Mach 5, the collisions of the gas molecules are no longer elastic (like balls on a pool table bouncing off each other), meaning that the ideal gas assumption becomes invalid. Instead, the gas particles slam into each other with such high energy that they begin to react. (Their kinetic energy is greater than the activation energy for a reaction to occur.) Additionally, in certain flight regimes, the vibrational excitation of the gas molecules decouples that energy mode from the rotational and translational energy, adding further complexity to the physics-based model. The final pathway for energy dissipation in hypersonic flow is through ionization. A large mass fraction of the electrons are stripped at very high Mach numbers (M>10) and altitudes (>60km). This phenomenology creates the plasma responsible for the communications black-out zone during re-entry. The graph below illustrates the regimes for different hypersonic flow physics and flight trajectories for known vehicles.
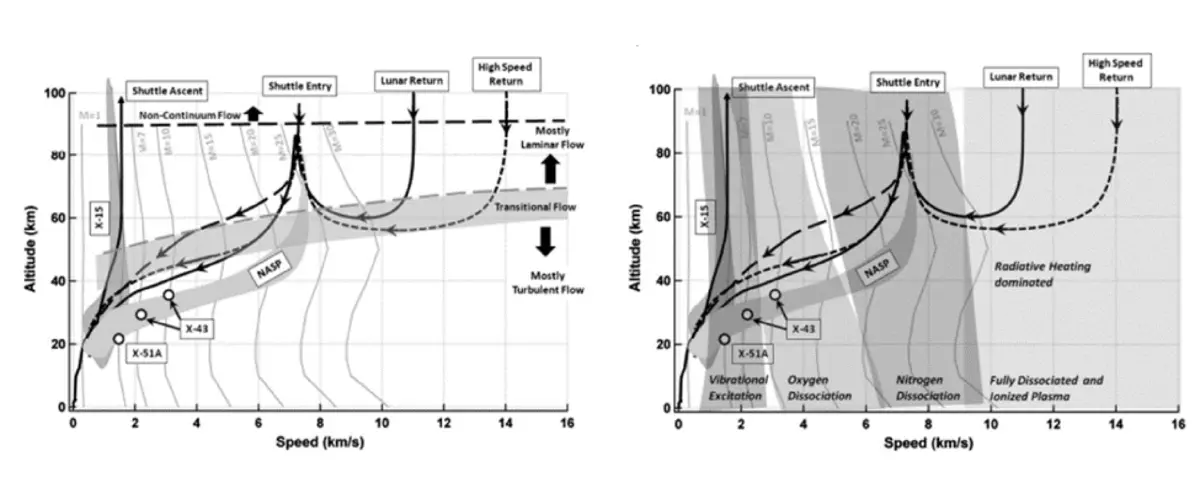
Hypersonic Flow Physics Phenomenology: (a) Turbulence Regime and (b) Thermochemistry[3]
These changes to the flow physics, known as thermochemical nonequilibrium flow, are a challenge to model accurately. Rather than write out the Navier-Stokes equations here, it’s faster to discuss their mathematical properties. The Navier-Stokes equations are mixed-type partial differential equations (PDEs). This means that the solutions are weak (non-unique) and dependent on the local flow properties. In the stagnation region of a hypersonic flow, they behave as elliptical PDEs, while beyond the Mach line they behave as hyperbolic PDEs. Getting a converged solution representative of reality is hard. That’s why test data and simplified approximations (aka hand-calcs) are so important — they anchor us to reality.
A key point to appreciate about the majority of our modern hypersonic computational fluid dynamics (CFD) solvers is that they all use a similar numerical approach to solve the equations: second order accurate finite volume implicit Reynolds Averaged Navier Stokes (RANS). This method does a decent job predicting steady-state integrated pressures for the vehicle, with uncertainties around +/- 8–10 percent, which is reasonably within experimental measurement uncertainty bands. It’s the heat flux predictions where they all suck, and they all suck together, to varying degrees of inaccuracy with an enormous range of uncertainties from -50 percent to +75 percent.[4]
One of the reasons all hypersonic CFD solvers have such large uncertainties on heat flux predictions is due to the lack of high-quality data in the flight regime. We are all still extrapolating our chemical reaction rates from 1960s shock tube data, because it’s the best data we have. (Tackling this source of epistemic uncertainty head-on, researchers are computing reaction coefficients from first principles statistical atomic collisions[5] and collecting new shock tube data.) The charts below illustrate the disconnect between ground test data we can acquire and the regimes where we actually fly.
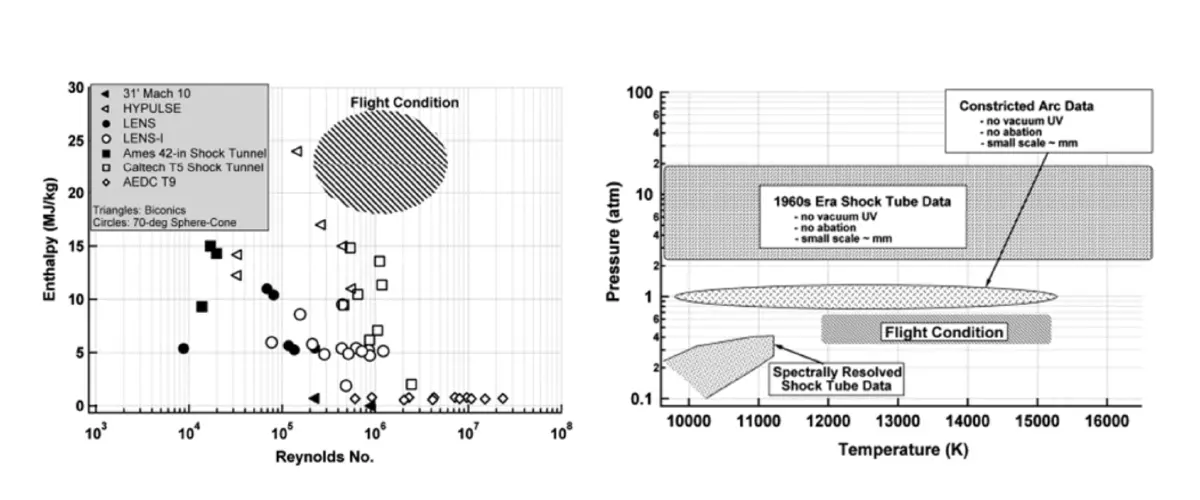
There’s not a ground-based wind tunnel on Earth that can replicate the conditions experienced in air during hypersonic flight. That’s part of what has slowed progress in this field – flight test is essential (and expensive)![3]
CFD practitioners create high-quality converged solutions by paying careful attention to the flow-field discretization (aka grid quality and timestep), boundary conditions, initial conditions, and selection of turbulence models appropriate for the flow regime. It’s very easy to create a garbage result using CFD!
Aerothermodynamic Design
In aerodynamic design, the function determines the form. In other words, aircraft look the way they do as a result of the flow physics they are required to fly through. Subsonic aircraft have soft rounded shapes with smooth continuous gradients in radius of curvature — the design intent is to keep the flow smooth and attached for as long as possible to reduce drag.
In contrast, supersonic aircraft have sharp leading edges. The design intent is to reduce drag by keeping the shock waves attached and as oblique as possible. The pressure drag dominates over viscous skin friction drag for supersonic aircraft, contributing ~85 percent of the total drag force. While aircraft designers optimize the outer mold line of the vehicle for cruise conditions, most supersonic aircraft also have variable geometry for the subsonic flight regime. Some famous examples: the Concorde’s articulating droop nose[6] and the F-14’s variable swept wings.[7] Of note, while the SR-71’s engine had linearly translating spike,[8] its purpose was to optimize supersonic flight performance by keeping the oblique shock attached to the engine inlet.
Moving on to aerothermodynamic design, what makes hypersonics hard is trying to survive the environment resultant from the heat flux scaling with velocity cubed.
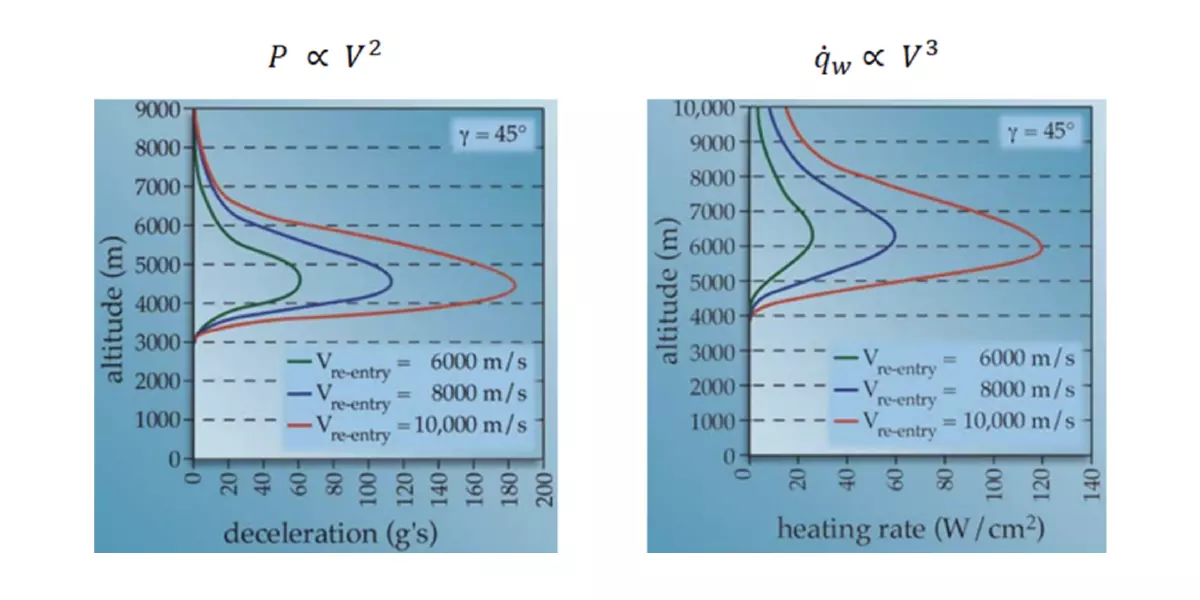
Force and heat flux on a blunt-body re-entry vehicle per FAA Guide Returning from Space[9]
Recap: subsonic vehicles have rounded edges and supersonic ones have sharp leading edges. Hypersonic vehicles go back to rounded (aka blunt) shapes. When designing a hypersonic vehicle, the top engineering priority shifts from reducing drag to surviving the heat loads. Blunt body theory was developed to push the shock wave off from the vehicle, as conventional materials cannot withstand the post-shock environment.
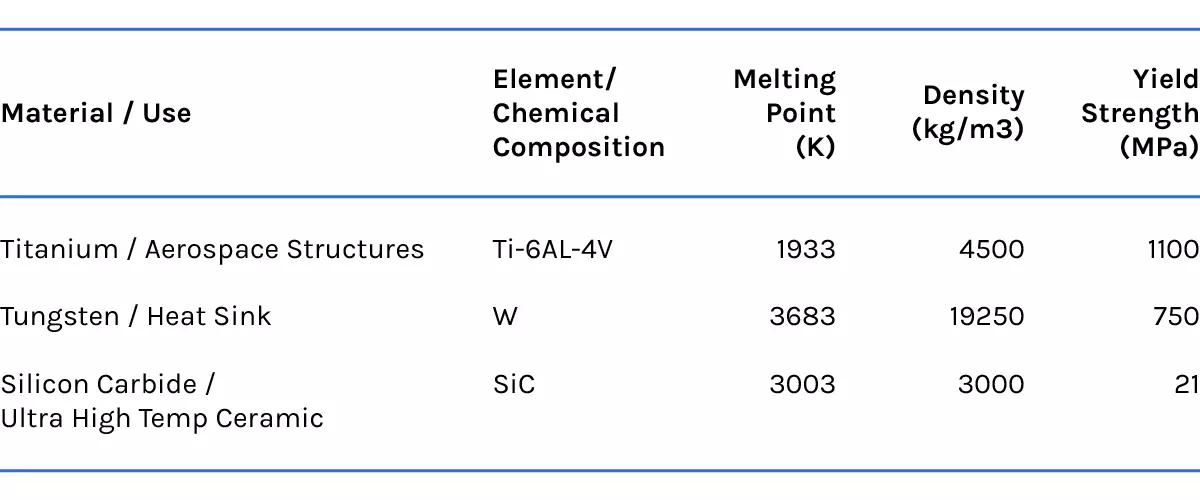
Aerospace materials, example use, and properties
Key takeaways from this table:
- Aerospace engineers love titanium because it’s light and strong, but its melting point is low.
- Tungsten has a much higher melting point, but it’s very heavy and somewhat brittle.
- Lots of ongoing research into using Silicon Carbide, among other types of Ultra High Temperature Ceramics (UHTCs), as a next-gen material for leading edges and as a coating layer on other parts of the vehicle. The problem with ceramics is their low fracture toughness and lack of tensile strength. (Impact resistance is a problem for all-ceramic thermal protection solutions, with the most tragic example being the loss of Shuttle Columbia.)
There are three types of thermal protection systems (TPS): passive, ablative, and active. Passive thermal systems are reusable; they rely on radiative heat rejection and low thermal conductivity materials (e.g. the Space Shuttle). Ablative thermal systems are not reusable, the heat load transfers into a material that pyrolyzes off during reentry (e.g. every manned reentry capsule to date). Active thermal systems circulate a fluid to reject the heat load — this is where promising technology development is ongoing for reusable hypersonic vehicles.
Synthesizing what we just learned about the hypersonic flow environment (it’s really hot), the ability to predict heat flux (CFD codes all suck by 50 percent or more), and the solutions available to withstand it — typical TPS designs will have a margin of 3x to ensure survivability of the vehicle in a worst-case scenario. These large TPS margins eat into the weight available to carry passengers and cargo. Hypersonic vehicle designers deal with the large uncertainties on predicted heat loads by allocating a substantial amount of their weight budget to thermal protection systems.
Hypersonics History
Imagine being nearly 100 years ahead of your time in predicting a technology — not just its existence, but the mechanisms for how that technology can be realized in practice. In their wonderful book Coming Home: Reentry and Recovery from Space,[10] NASA historians Roger Launius and Dennis Jenkins briefly cover one of the earliest concepts for a hypersonic spaceplane: the Sänger-Bredt Silverbird. While getting his PhD at the Vienna Polytechnic Institute in 1929, Eugen Sänger conceptualized a reusable rocket-powered space plane that would fly in a phugoid motion (skip-gliding along the top of the atmosphere). He would later refine the design with his mathematician wife Irene Bredt. The design reference trajectory and method of propulsion were both feasible back then (and variations of this solution architecture are used in military hypersonic systems today). What Sänger underestimated were the heat loads.
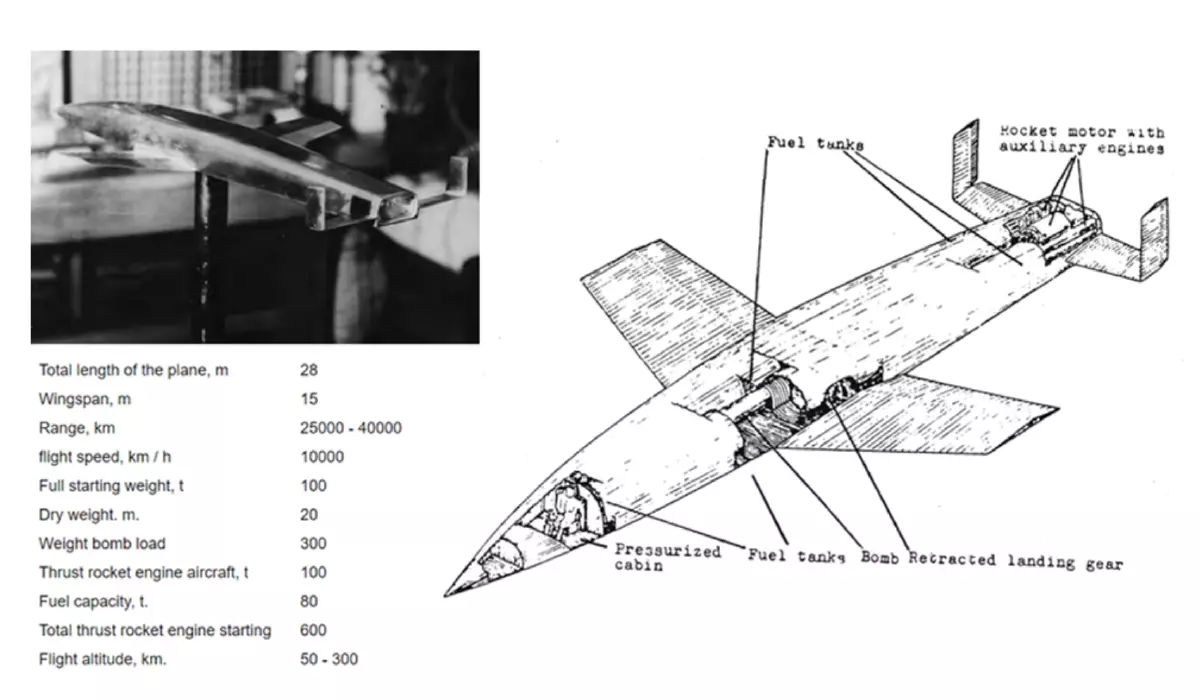
Upper left: a wind tunnel model for the Silverbird,[10] Lower left: a summary table of the Silverbird conceptual design specifications,[11] Right: a Sänger-Bredt report drawing[12]
There exists somewhat of a debate as to the identity of the first vehicle to achieve hypersonic speeds. As aerospace legend John Anderson notes for The Smithsonian,[13] the first object to travel hypersonic (over 5 times faster than the speed of sound) was in 1949, via a ballistic trajectory. The first piloted aircraft to achieve hypersonic flight was the X-15, NASA’s rocket-powered test vehicle,[14] whose first flight occurred in 1960. (Fun fact: Neil Armstrong was an X-15 pilot before joining the Apollo program.)
Other notable milestones for hypersonic flight include NASA’s X-43,[15] which was the first scramjet propulsion system to demonstrate net-positive thrust. A scramjet, aka a supersonic combustion ramjet, is an air-breathing propulsion technology that enables an order-of-magnitude higher fuel efficiency than a chemical rocket. (Rockets take BOTH propellants with them — fuel and oxidizer. Jet engines bring along the fuel and combust it with air they intake during flight.) The X-43A flights occurred in 2004, achieving a top speed of Mach 9.6 and a total of 10 seconds of engine operation time.
The next major milestone for hypersonic flight was accomplished by the Air Force’s X-51A flight in 2010.[16] The X-51A demonstrated net-positive thrust for a hydrocarbon-fueled scramjet, burning for over 200 seconds at Mach 5. The important distinctions between hydrogen as a scramjet fuel and conventional hydrocarbon jet fuel are: (1) hydrogen has a much higher flame speed thus is easier to light in a supersonic combustion environment and (2) hydrogen is more energetic. The X-51A achieving sustained net-positive thrust with a hydrocarbon scramjet was the hypersonic equivalent of the Wright Brothers’ Flight.
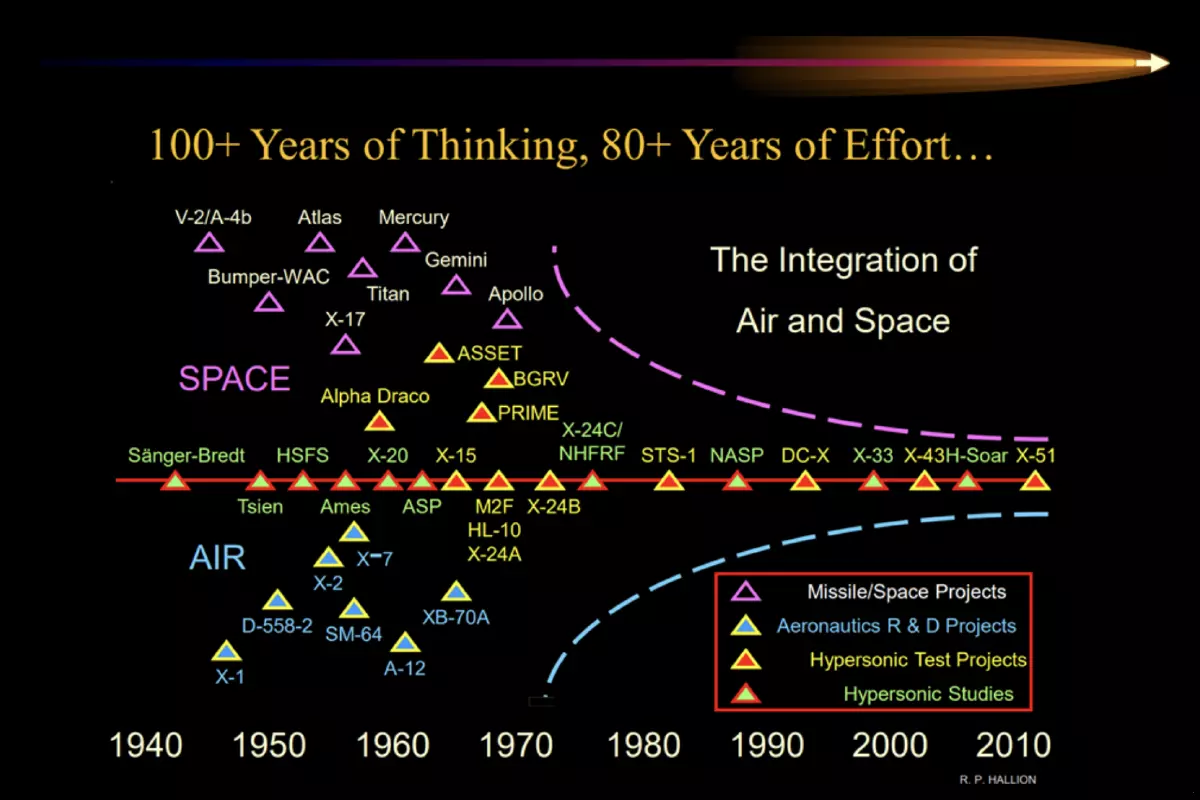
The history of hypersonics research. (Special thanks to Dr. Richard Hallion for sharing his latest version of this graphic!)
Observant readers will note a ~40-year gap between the X-15 and the X-43 programs for successfully demonstrated hypersonics milestones. Hypersonics development in the U.S. was cyclical for decades. Beyond the lack of an external impetus to develop hypersonic capabilities, another reason that U.S. funding for hypersonics came in fits and starts was due to some high-cost, high-profile failures. Depending on who you ask, there are many documented reasons as to why the National Aero-Space Plane (NASP) failed. (By the time NASP was canceled in 1992 it was 500 percent over budget and 11 years behind schedule.) Until a few years ago, I had assumed the heat exchanger technology was the main reason NASP failed. In 2019, I attended a fantastic lecture by Dr. Richard Hallion where he shared a story about the programmatic failures of NASP — constantly changing requirements that grew ever more ambitious — aka “death by scope creep.”
Clear, well-defined requirements that don’t change are just as important to success as understanding the relevant fundamental physics. Engineers can’t design a bleeding-edge solution for problems as challenging as those endemic to hypersonics if the requirements are ill-defined and continually changing. This alone drives one reason to remain optimistic about non-traditional commercial hypersonic companies: they are setting their own requirements for the product, rather than the inefficient and painful iterative process with government program managers that happens today.
Economics of the Concorde
The last time civilians were able to fly supersonic speeds for commercial air travel was in 2003, when the Concorde retired. For 27 years the Concorde carried 100 first-class passengers, cruising at Mach 2 across the Atlantic Ocean.
While the Concorde was a commercial jet, it was heavily subsidized by the British and French governments due to its operational economics. The subsonic takeoff required after-burning engines with 215 percent higher fuel burn than in supersonic cruise![17] By contrast, subsonic high-bypass turbofan engines of that era (eg CF6-80C2) had 217 percent better fuel efficiency in cruise. At take-off the Concorde burned 4x more fuel than a subsonic jet! The Rolls Royce/SNECMA Olympus 593 engine afterburners were throwing 2x more fuel into the back for only 19 percent more thrust. (It’s worth noting the North American Xb-70 Valkyrie had GE YJ93 engines that were more fuel efficient and enabled supersonic cruise at Mach 3.) The unoptimized aerodynamics and underperforming engine limited the Concorde’s range to 3900 nm, constraining operations to transatlantic flights only.
There were plans for an improved B iteration of the Concorde, with morphing leading edges on the wings that could improve take-off lift/drag ratio by ~8 percent, removing the after-burner requirement. The improved aerodynamics and engine efficiency would have increased range by ~15 percent, allowing transpacific routes. However, the Concorde’s manufacturers were having difficulty selling the remainder of their original production run. An improved Concorde, with better operational dynamics, was never produced.
In the 1960s there were 74 orders for the Concorde aircraft from 16 different airlines. By the time the aircraft was ready to enter service in the 1970s, only the two national carriers, British Airways and Air France, took fulfillment of their contract, ordering nine aircraft total. The remaining five Concordes built were subsidized, and production ended in 1980. (It was estimated a production run of 100 Concordes would be needed to get a reasonable return on the R&D costs for the design. In 1977 the unit cost of a Concorde was £23 million.[18])
The factors contributing to this dramatic drop in demand included: (1) no penalty for cancellations, (2) the 1973 OPEC oil embargo increased the price of fuel 4x, and (3) 1976 overland sonic boom restrictions killed many routes. Due to the oil crisis the airline operational trend shifted from high speed to high capacity wide-body subsonic jets like the 747.
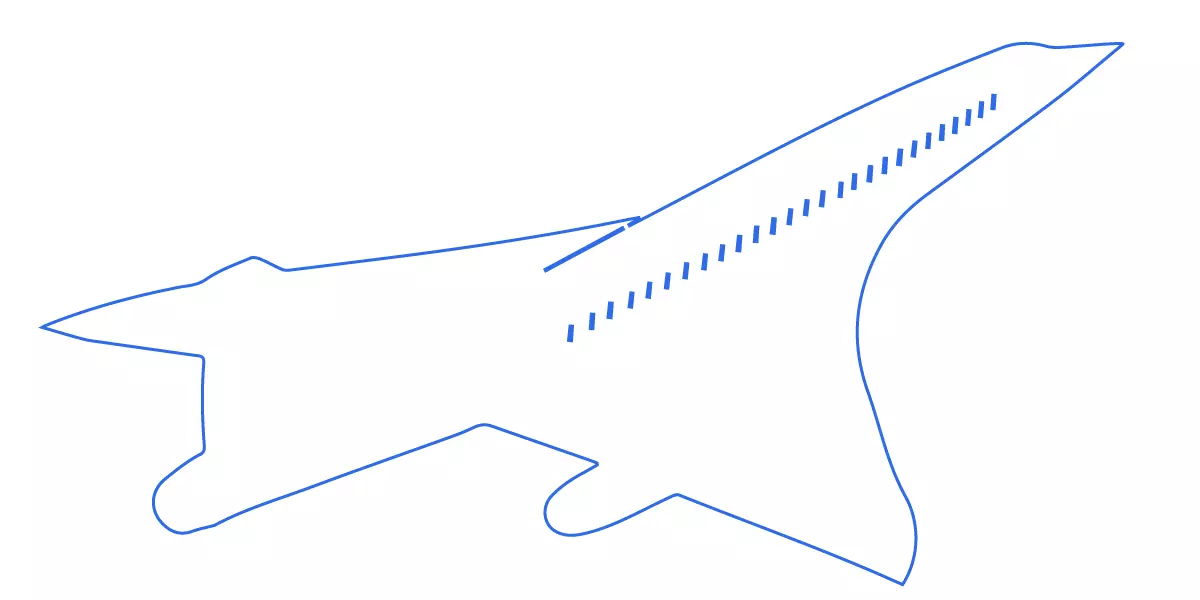
Politics Kills Progress
The Concorde was not the only commercial supersonic jet of its era. The desire for a supersonic commercial jet became a national prestige project for the Americans, the Russians, and the British and the French (who partnered together via treaty). In fact, the Soviet Union Tupolev Tu-144 was actually the first to enter commercial service in December 1975 (Concorde entered service January 1976), but it flew for a limited career of 30 months, due to design flaws that caused two fatal crashes. The American Supersonic Transport (SST) program saw competition from five companies: Convair, Douglas, North American, Lockheed, and Boeing. Yet an American SST was never built.
Depending on whose book you read, Conway’s High-Speed Dreams[19] or Simons’ Valkyrie XB-70,[20] American President John F. Kennedy had a rivalry with French President Charles de Gaulle around commercial supersonic jets. De Gaulle campaigned on restoring French greatness in the world by making France technologically independent from all other countries, focusing his efforts on nuclear power and aerospace. Both books agree on the impetus for the American SST program being national prestige, but differ on whether JFK would spend whatever it took to win.
The two books also offer contrasting opinions of Secretary of Defense Robert McNamara, who was ultimately responsible for ending the American SST program. Either McNamara was hell-bent on killing the XB-70 and the American SST because of a back-of-the-envelope calculation he did, or he was an early student of systems engineering who wanted the program closely studied to verify or refute his hand calc.
Originally the SST program was under the FAA, and had proposed a 75/25 funding split whereby the government would pick up the larger portion of the R&D budget, but the U.S. companies participating were expected to contribute a decent amount of private risk capital. (The estimated program cost of $1B.) JFK commissioned an independent review of the aircraft industry’s financial capacity; the three CEOs who were interviewed (from Lockheed, Boeing, and North American) all wanted the cost-share to be reduced to 10 percent. This lack of desire for real skin-in-the-game convinced McNamara the airframers didn’t believe in the financial viability of a supersonic commercial jet.
The final political blow to supersonic transport came in 1976 with the overland ban of sonic booms. A recent IEEE Spectrum article does a great job covering the history behind the FAA’s sonic boom noise regulations.[22] To summarize here for brevity: overland supersonic flight has been banned in the U.S. since 1973. The FAA’s regulatory ban was informed by the extensive flight testing they completed a decade prior. In 1964, citizens of Oklahoma City experienced 8 sonic booms per day for nearly 6 months. The FAA’s study of ~1,200 flights garnered 15,000+ noise complaints and damaged property claims (cracked brittle materials); 25 percent of the Oklahoma City population was not in favor of the sonic boom noise. If the FAA had run a more reasonable campaign, perhaps the anti-SST movement would have never taken off.
Enabling Technology: Sonic Boom Suppression
With our background tour of the fundamentals and history complete, we’re ready to dive into the state-of-the-art research on aerodynamic design for sonic boom suppression. To recap, we learned earlier that there is an abrupt pressure rise behind a shockwave — the birthplace of the sonic boom. (Sound is a pressure wave.[23]) Flying at a higher altitude, in less dense air, reduces the sonic boom by reducing the absolute pressure and increasing the path length for sound energy to dissipate on its way to the ground.
A normal shock is the strongest discontinuity that can be created at a given speed — the more oblique the shockwave the less its strength and thus the resultant sound. This translates to a long pointy outer mold line for low-boom supersonic passenger aircraft. That guiding principle is used on both NASA’s X-59 design[24] and JAXA’s D-SEND2 research aircraft.[25] Supersonic aircraft designers also minimize the strength of the shockwaves that propagate towards the ground by placing features that can disrupt flow, such as engine inlets, on the upper surface of the vehicle. For completeness' sake, long and pointy isn’t the only design paradigm for low-boom design. There is an MIT biplane concept that reduces noise by canceling out shocks.[26]
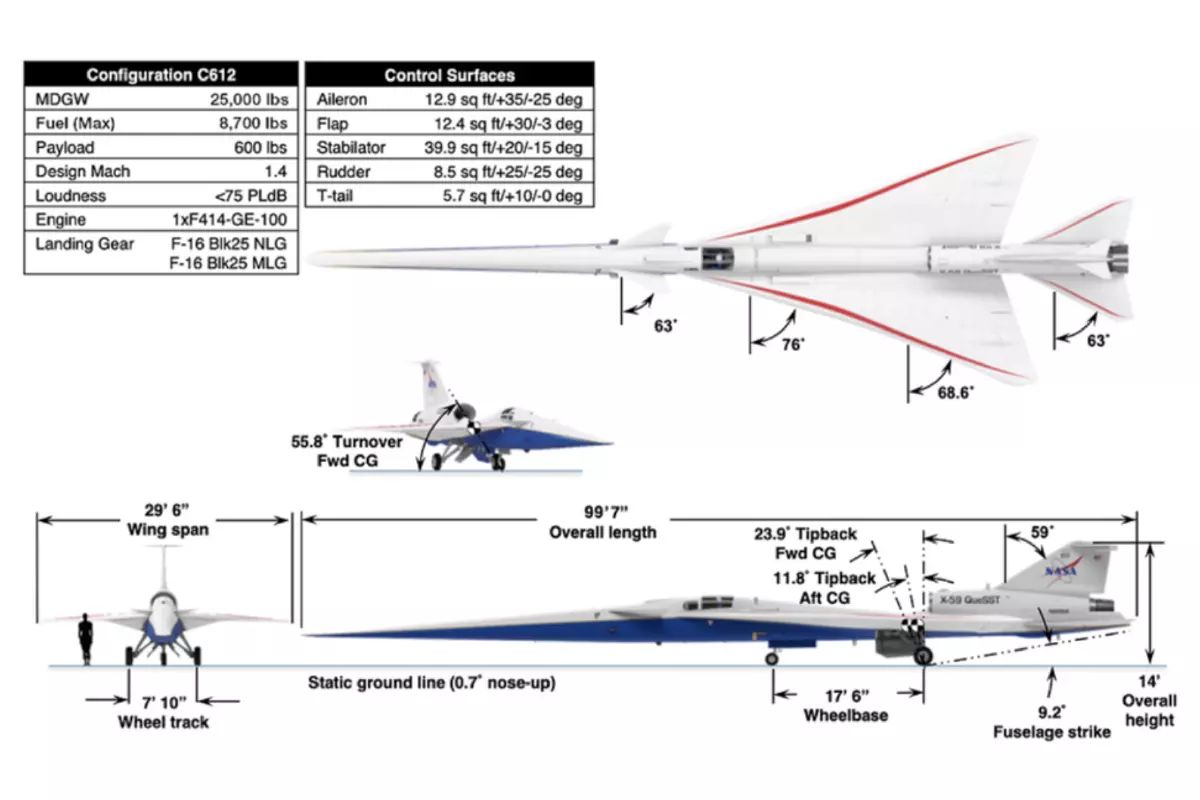
NASA’s X-59 QueSST[27]
The goal of NASA’s quiet-boom demonstrator was not to make the quietest design for that size of aircraft, but rather to create a supersonic demonstrator that can provide the ground noise data necessary to re-write the rules for overland supersonic travel.
The X-59’s design goal for ground perceived noise is 75 decibels, roughly 8 times quieter than the Concorde. (Average urban background ambient noise levels are between 60–70 dB.[28]) As seen in the below images, the X-59 is predicted to meet its design requirement across its entire sonic boom ground footprint.
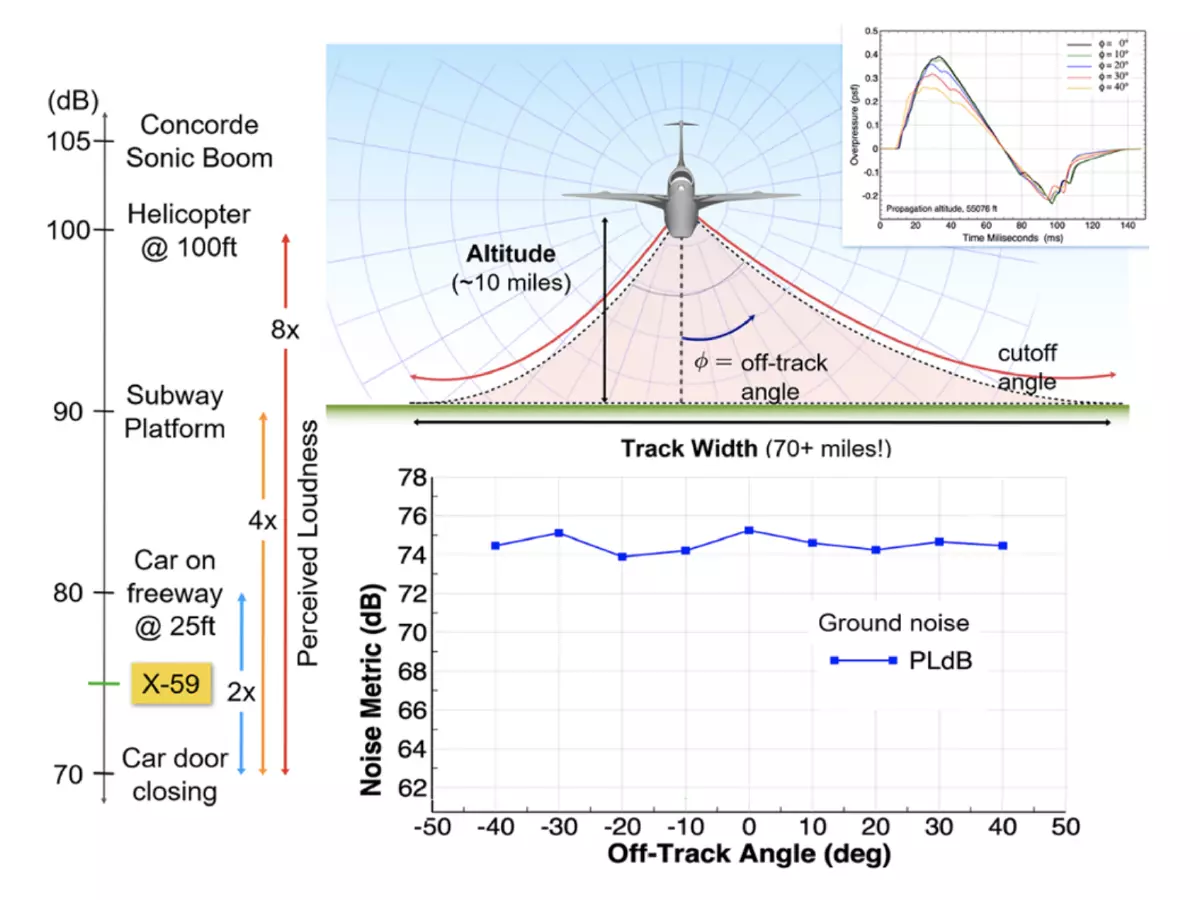
The X-59 has been designed to leave a sonic thump over it’s 70mile wide boom carpet[29]
The X-59 is scheduled to begin flying in 2022, with the critical community noise data being collected starting in 2024.[30] The flight tests will also provide data to help validate simulation tools and put experimental error bars around the design predictions. Knowing the pre-test accuracy of modeling and simulation results enables supersonic aircraft designers to scale up the low-boom design principles for large passenger vehicles with sufficient design margin for success.
Hypersonic Enabling Technologies
The most exciting innovations for hypersonics are happening in propulsion and active thermal management systems for reusable hypersonic vehicles. U.K. company Reaction Engines has the SABRE engine, which uses a novel air-breathing rocket combined cycle. In 2015, the Air Force Research Lab independently validated the SABRE engine’s thermodynamic cycle.[31] (This author loves a good closed-loop regeneratively driven turbine cycle!) Reaction Engines also has heat exchanger technology they validated in 2019 under a full-scale testing program funded by DARPA.[32] Another approach to hypersonic propulsion technology is being taken by Atlanta-based startup Hermeus, which is using a turbine ramjet combined cycle for their propulsion system. While the turbine-based combined cycle (TBCC) is not novel, stable ‘mode transition’ between turbine engine and ramjet operations at hypersonic speeds will be a major technical breakthrough. (In 2016, DARPA funded the Advanced Full Range Engine (AFRE) program for a hypersonic TBCC demonstrator engine.[33] There is no public information on the result of the AFRE program.) Prime Movers Lab portfolio company Venus Aerospace is working on their own novel approach to propulsion technology and active cooling. I wish I could share more at this time! Like all Prime Movers Lab companies, they are leveraging a recent scientific breakthrough for their key technology.
In an earlier section of this paper, the flight demonstration of scramjet combustors was hailed as hypersonics’ Kitty Hawk moment. Yet none of the startups covered in the prior paragraph are focused on scramjets for their propulsion solution. This is because current scramjet designs are constrained in size and operational envelope due to the combustion dynamics at supersonic speeds. The challenge to overcome is that supersonic combustion dynamics is mixing limited.[34] To improve scalability and operability, clever engineers are working on solutions that improve mixing efficiency while maintaining reusability and without substantially increasing pressure losses. Two promising research approaches to watch come from University of Texas Arlington[35] and FGC Plasma. (It’s worth noting that Australian startup Hypersonix has a scramjet design and is partnered with Boeing[36] and with Kratos[37] on military applications.)
What about breakthroughs in materials? For systems that are not reusable, the most exciting innovation in heat shield materials comes from NASA’s 3-D carbon fiber woven HEEET material,[38] which will enable capsules to return humans safely from Mars. For passive thermal management systems, there is research being done on additive manufacturing for Ultra High-Temperature Ceramic structures,[39-42] to enable hybrid material architectures that provide more robust properties.
These new hypersonic technologies, combined with modern multi-disciplinary engineering design and optimization tools, are enabling humans to push the envelope of what’s possible for high-speed flight. In fact, the sum of these advances is what recently emerged from stealth-mode startup Radian is pushing for their single stage to orbit (SSTO) space plane.[43] Chinese startup Beijing Lingkong Tianxing Technology is also entering the spaceplane race, having received nearly $63M in funding last year.[44]
Commercial Market
The commercial supersonic flight market can be divided into two segments: smaller-sized business jets that carry less than 20 passengers and larger-sized commercial passenger jets that carry over 50 passengers.
There are currently two entrants to the larger passenger jet supersonic commercial market: Boom and Exosonic. Boom, a Prime Movers Growth portfolio company, has a commanding lead in this market segment, as evidenced by the 65-seat to 88-seat Overture’s design maturity and their recent order with United to enter into service in 2029.[45] Boom has also made tangible technical progress, reducing risk through its XB-1 demonstrator airplane, scheduled to fly in 2022.
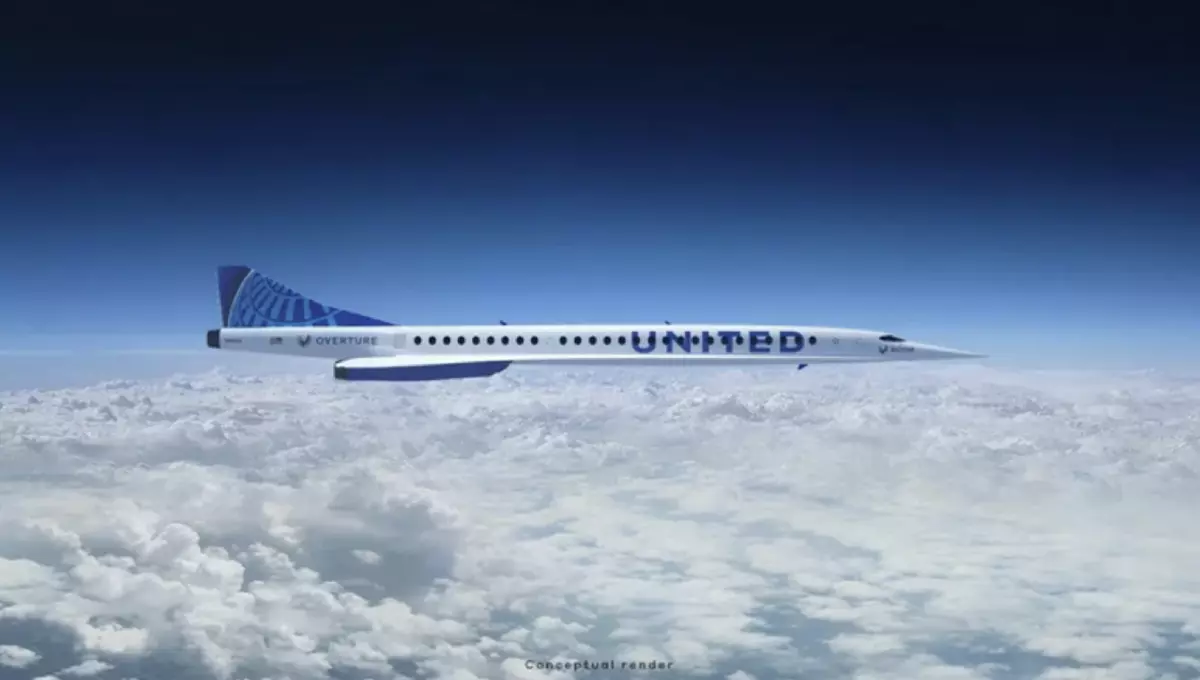
United placed an order for 15 Overture aircraft from Boom[45]
Exosonic, the newest entrant to the commercial supersonic market, has a 70-seat low-boom conceptual design. Exosonic graduated from Y Combinator in March 2020 with $150K of funding and was awarded a $450K STTR grant to collaborate with Stanford on transitioning their design for a military cargo application.[46] Whether Exosonic can leverage their initial work into a larger program, as both Boom and hypersonic startup Hermeus recently did with their $30M STRATFI DOD awards, remains to be seen.
Building aircraft is a capital-intensive business. Despite a $10B backlog of orders for 300 of its low-boom 12-passenger business jets, Aerion Supersonic closed in May 2021.[47] There are two remaining entrants for the supersonic business jet market: Spike Aerospace and Virgin Galactic. Spike Aerospace has a mature 18-passenger low-boom design, which they are aiming for market entry in 2028, targeting lucrative routes overland in markets such as Asia.[48] Meanwhile, in August 2020, Virgin Galactic announced its plans to develop a 19-passenger low-boom business jet,[49] partnering with Rolls Royce for the propulsion and NASA for the aerodynamic design.
In 2020 NASA funded high-speed flight commercial market studies that are all available for free on the NASA Technical Reports Server.[50-53] If you’re ever daydreaming of better travel while on a long-haul subsonic flight, peruse these reports to understand the dynamics driving a faster future. Both groups did wonderful work analyzing the market, business case, and barriers. The first two charts I’d like to highlight from those reports illustrate the time savings by speed class and the customer demand signal for the value of that saved time from surveyed high net-worth individuals.
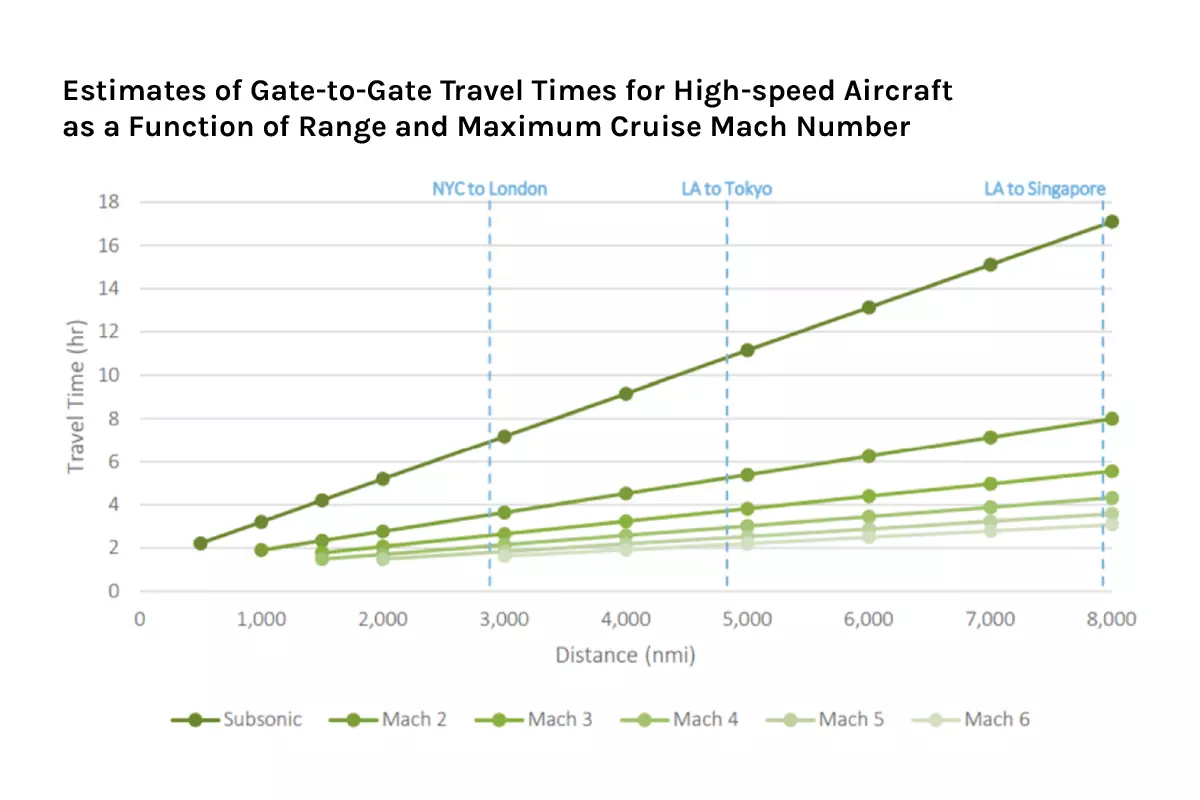
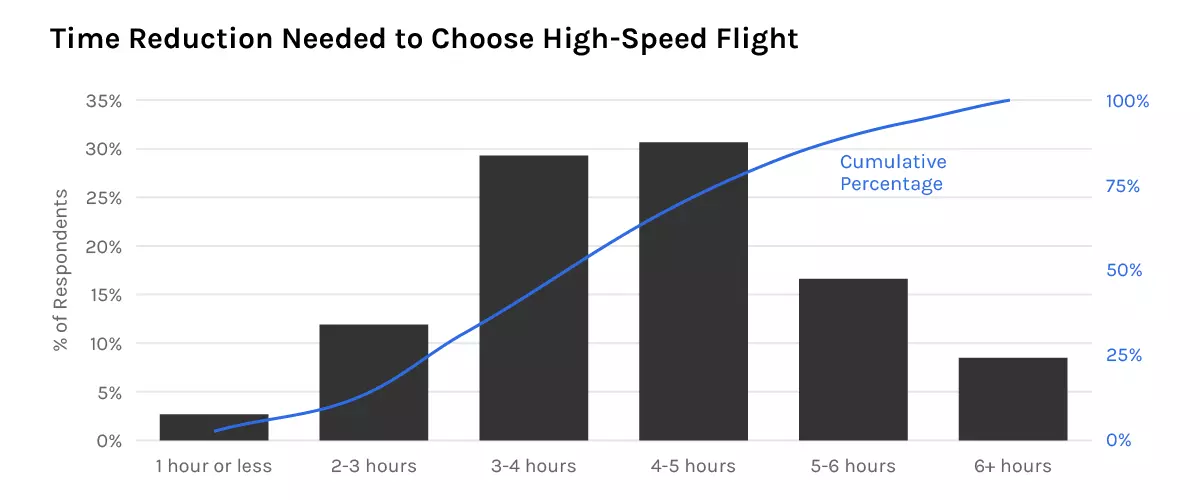
Upper image: High-Speed Flight “Shrinks” the World. Time savings over a distance for different cruise Mach numbers.[52] Lower image: Value of Time. Results from a survey of 150 high-net-worth individuals (70% of survey respondents took more than one 5-hr flight annually).[51]
What stands out from these charts is that the nautical range for city pairs is just as important a characteristic for a high-speed commercial transport as the speed at which a vehicle flies. Supersonic jets hit the demand sweet spot for both Atlantic and Pacific routes. Commercial hypersonic jets have the opportunity to differentiate themselves along the Pacific routes. Based on the above charts, Prime Movers Growth portfolio company Boom has a well-positioned product with their supersonic Overture aircraft.
The work done by Deloitte/SpaceWorks/NIA utilizes a conceptual aircraft design and cost modeling tool developed by SpaceWorks called ROSETTA. The following charts show the Internal Rate of Return for different high-speed aircraft. A notable conclusion from both reports is that the business case for high-speed flight exists without government subsidies.
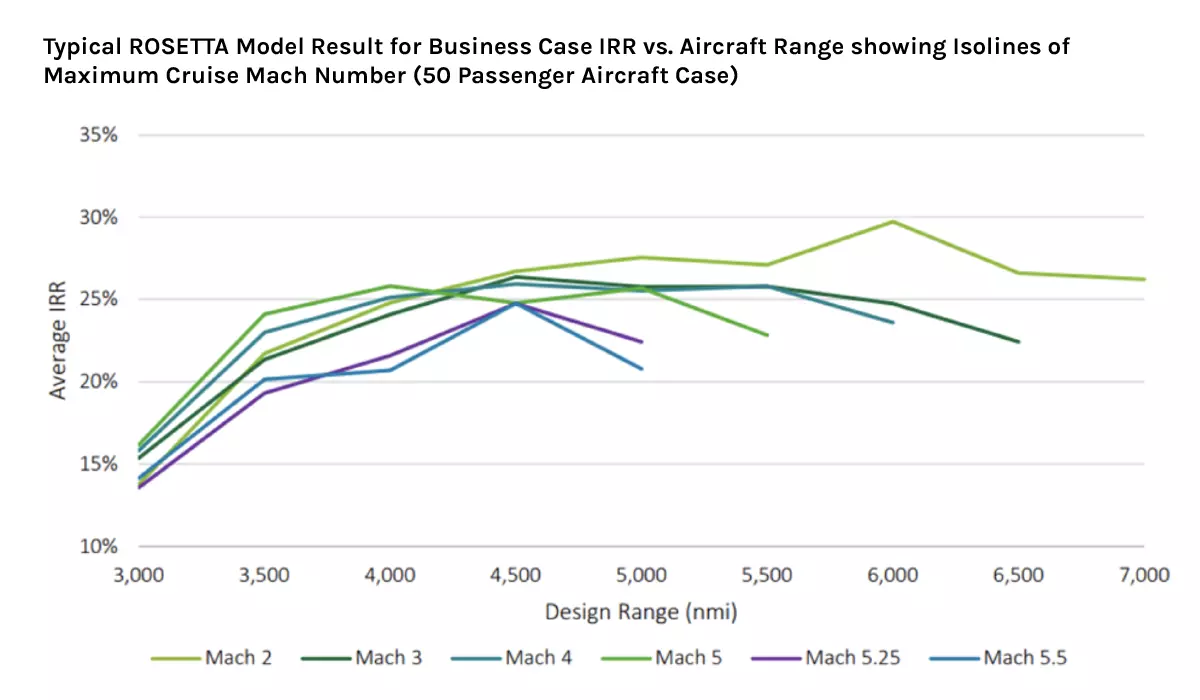
High-Speed aircraft can operate profitably thanks to factors like improvements in engine efficiency and aerodynamic design optimization. SpaceWorks ROSETTA model accounts for aircraft design changes needed for different flight regimes (supersonic vs hypersonic).[53]
The BryceTech/SAIC report has a great chart illustrating the high-speed flight market landscape. (Note: this study was done before Prime Movers Lab portfolio company Venus Aerospace went out of stealth, so they do not appear in the chart. Their range and propellant would spot them with the class of vehicles described in Case 5 below.)
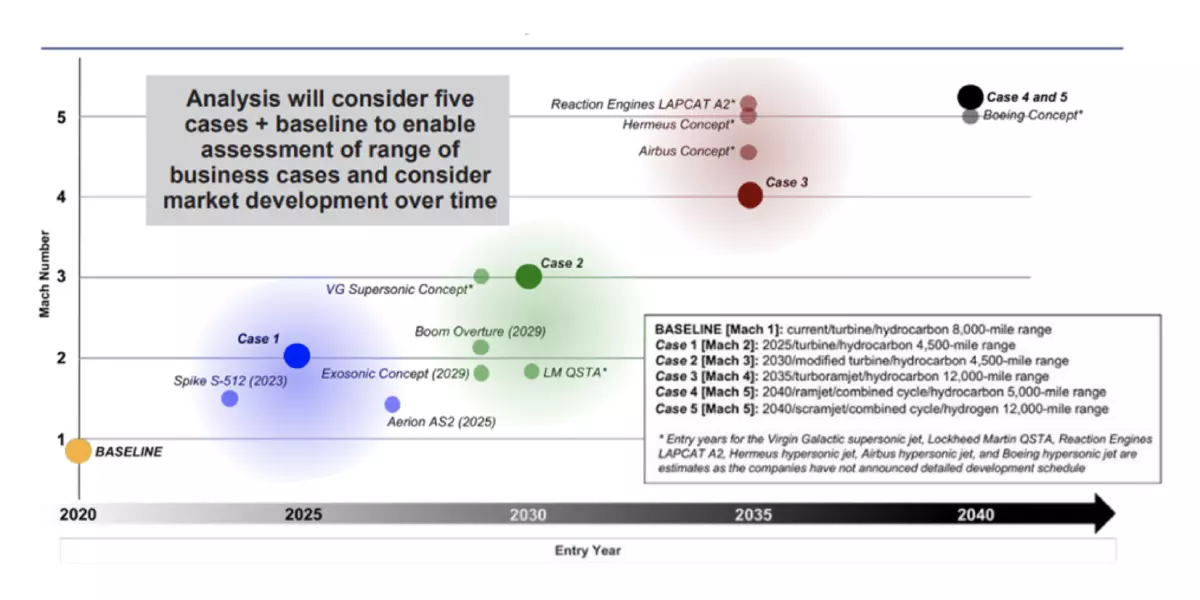
High-Speed flight landscape as of 2020. Concepts from Venus Aerospace, Hypersonix, and Destinus would fall under Case5 definition for hydrogen-fueled vehicles.[50]
One reason NASA ordered these market studies was to solicit outside feedback on what they could do to help remove barriers for the commercial hypersonics aircraft companies. NASA wanted to understand the customer demand for high-speed flight, the pressures on the business case, and any non-technical roadblocks (e.g. regulations). Both reports tested sensitivity to government R&D spend and government purchase of a certain minimum number of hypersonic aircraft. The good news for hypersonic commercial aircraft companies is that neither is required. The better news is that the US government will continue to invest heavily into hypersonic R&D because of the defensive applications.
Dual-Use Technologies Increase TAM
Human-rated high-performance aerospace vehicles tend to follow a development path that starts with defense applications and transitions to civil applications as technology matures and becomes commonplace. The Mercury 7, carrying America’s first astronauts, rode to space atop modified ICBMs in the 1960s. Dual-use technologies are defined as those that have both civilian/commercial and defensive applications.
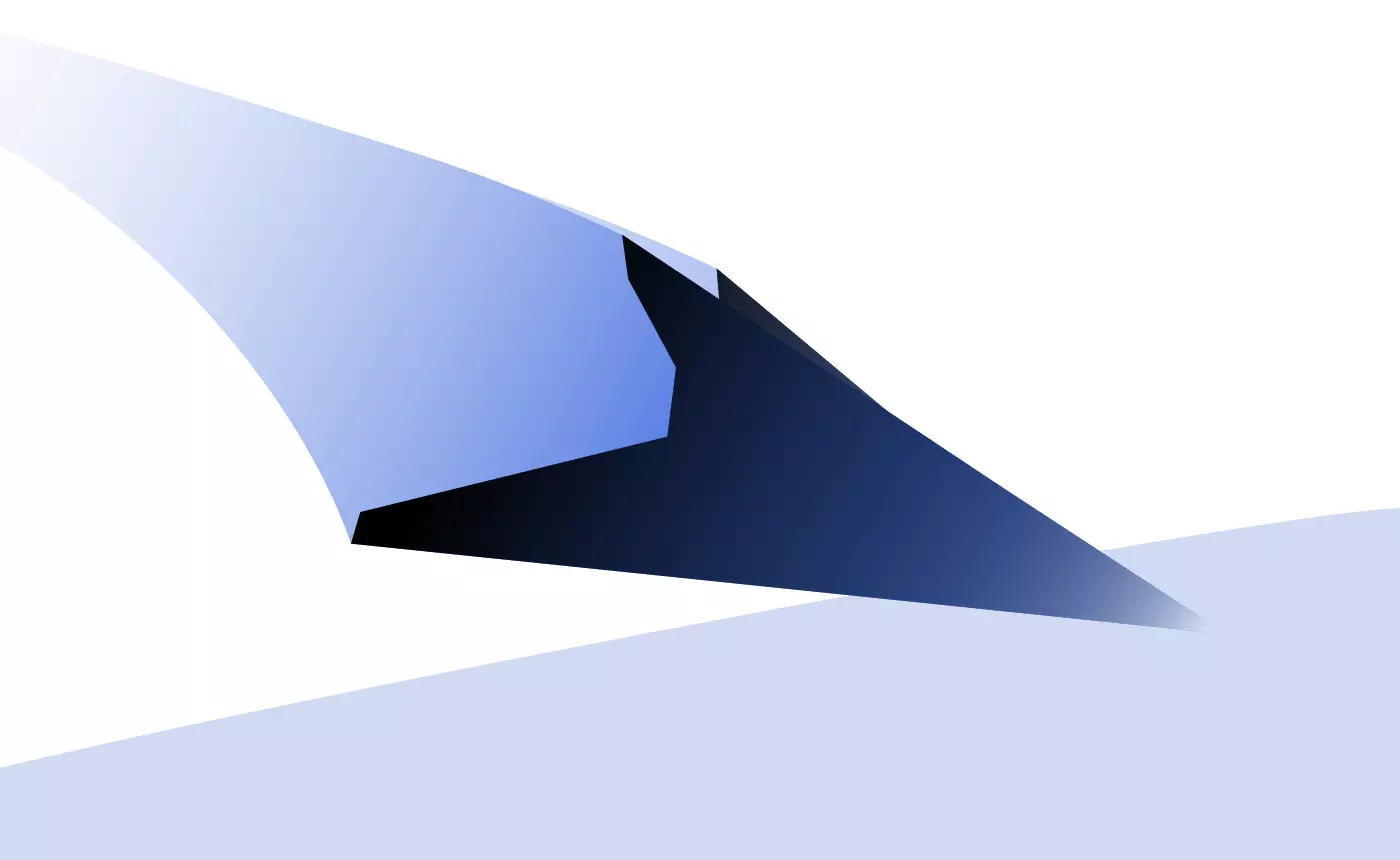
To properly understand the context for why hypersonics is here to stay, it’s necessary to evaluate the defense landscape. In the 2018 National Defense Strategy[54] the United States turned its focus away from the War on Terror, and towards the reality of a 21st century Great Power Competition. Unlike the Cold War, the United States now has two opposing near-peer competitors, the Chinese Communist Party and the current Russian government. Our adversaries have tested and fielded hypersonic systems, as summarized on pages 12–16 in a briefing on hypersonics for Congress.[55]
Why are hypersonic capabilities critical to continued U.S. security? The hypersonics advantage for military applications is rooted in the combination of speed plus maneuverability. The maneuverability of a hypersonic weapon creates a wide uncertainty band around its trajectory: these things are very hard to shoot down! The United States has demonstrated the ability to intercept ballistic missiles. Defense against hypersonics is an ongoing area of active research. For more reading on why hypersonics are critical to our future defense, please read the 2021 piece by the Pentagon’s Head of R&D for hypersonics, Mike White, in Breaking Defense.[56]
Dual-use technologies can be a way to reduce market risk. If the developed technology can also be used for defense applications, the business case for these hypersonic aircraft developers gets much stronger.
Companies to Track
Boom
Location: USA
Products: Commercial supersonic jet
Propulsion: Low-bypass turbofan
Partnerships: Air Force
Exosonic
Location: USA
Products: Commercial supersonic jet
Propulsion: Low-bypass turbofan
Partnerships: Air Force
Spike
Location: USA
Products: Supersonic private jet
Propulsion: Low-bypass turbofan
Partnerships: N/A
Hermeus
Location: USA
Products: Reusable hypersonic aircraft
Propulsion: Turbine-based combined cycle
Partnerships: Air Force
Venus
Location: USA
Products: Reusable hypersonic aircraft
Propulsion: Still in stealth-mode
Partnerships: DARPA, NASA
Radian
Location: USA
Products: Sled-lauchned SSTO space plane
Propulsion: Rocket
Partnerships: ?
FGC Plasma
Location: USA
Products: Tactical missiles, SAF compatible igniters
Propulsion: Scramjet
Partnerships: Air Force, DARPA, DOE
Reaction Engines
Location: UK
Products: Heat exchanger, SABRE engine
Propulsion: Rocket-based combined cycle
Partnerships: Air Force, Ministry of Defense
Hypersonix
Location: Australia
Products: Military drone, spaceplane
Propulsion: Scramjet
Partnerships: Boeing, Kratos
Beijing Lingkong Tianxing Technology
Location: China
Products: Reusable hypersonic aircraft
Propulsion: ?
Partnerships: N/A
Conclusion
At Prime Movers Lab we believe in the power of human connection and the precious value of time. High-speed flight is no longer a game of national prestige, subject to the whims of politics. It’s become the domain of private industry, where the technology is mature enough that entrepreneurs can focus on designs that reduce business risk. In the next decade we anticipate commercial high-speed flight will return to the market, regulations around overland sonic boom will be changed thanks to NASA’s X-59 program, and hypersonic technologies will transition from military to civilian flight. The future is faster!
References
[1] Shapiro, A., The Dynamics and Thermodynamics of Compressible Fluid Flow, p. 122, 1953
[2] Candler, G., Rate Effects in Hypersonic Flows, Annual Review of Fluid Mechanics, 2019
[3] Bose, D., et. al., Uncertainty Assessment of Hypersonic Aerothermodynamics Prediction Capabilities, Journal of Spacecraft and Rockets, Vol 50, No. 1, 2013
[4] Bose, D., et. al. , Uncertainty Assessment of Hypersonic Aerothermodynamic Prediction Capability, 42nd AIAA Thermophysics Conference, Honolulu HI, June 2011, AIAA-2011-3141
[5] Chaudry, R., et. al., Quasiclassical Trajectory Analysis of Oxygen Dissociation via O2, O, and N2, AIAA SciTech Conference, Kissimmee FL, Jan 2018, AIAA-2018-0237
[6] www.concordesst.com/nose.html
[7] Kress, R., Variable Sweep Wing Design, Aircraft Prototype and Technology Demonstrator Symposium, Dayton OH, March 1983, AIAA-83-1051
[8] www.enginehistory.org/Convention/2014/SR-71Inlts/SR-71Inlts.shtml
[9] FAA Guide, Returning from Space
[10] Launius, R., and Jenkins, D., Coming Home: Reentry and Recovery from Space, NASA SP-2011-593
[11] www.globalsecurity.org/wmd/world/germany/sanger.htm
[12] https://apps.dtic.mil/sti/pdfs/AD1082458.pdf
[13] airandspace.si.edu/stories/editorial/hypersonic-flight
[14] history.nasa.gov/SP-60/ch-1.html
[15] www.nasa.gov/centers/dryden/history/pastprojects/HyperX/index.html
[16] www.af.mil/About-Us/Fact-Sheets/Display/Article/104467/x-51a-waverider
[17] www.heritageconcorde.com/concorde-olympus-593-mk610-engines
[18] Buttler, T., and Carbonel, J.C., Building Concorde: From Drawing Board to Mach 2, Ch. 10, 2018
[19] Conway, E., High-Speed Dreams: NASA and the Technopolitics of Supersonic Transportation, 1945-1999, 2008
[20] Simons, G., Valkyrie The North American XB-70: The USA’s Ill-fated Supersonic Heavy Bomber, 2011
[21] McGinn, J., and Lofgren, E., Three steps to help defense innovation break free from its shackles, Defense News, Jan 2022
[22] Schneider, D., The New Supersonic Boom, IEEE Spectrum, Aug 2021
[23] www.nasa.gov/specials/X59/science-of-sound.html
[24] www.nasa.gov/specials/X59
[25] www.aero.jaxa.jp/eng/research/frontier/sst/d-send.html
[26] https://news.mit.edu/2012/supersonic-biplane-0319
[27] www.nasa.gov/aero/x-59-quesst-overview
[28] www.noisequest.psu.edu/noisebasics-basics.html
[29] Nemec, M., Aftosmis, M., and Spurlock, W., Minimizing Sonic Boom Through Simulation-Based Design: the X-59 Airplane, SC19, Denver, CO, Nov 17–22, 2019
[30] www.nasa.gov/specials/X59/whats-next-for-x59.html
[31] www.airforce-technology.com/news/newsafrl-confirms-feasibility-of-rels-sabre-engine-concept-4558439
[33] www.darpa.mil/news-events/2016-06-24
[34] Drummond, J.P., Diskin, G.S., and Cutler, A.D., Fuel-Air Mixing and Combustion in Scramjets, 2006
[35] Vigano, D., and Maddalena, L., On Sustaining Turbulence Production in Interacting Supersonic Streamwise Vortices, Physics of Fluids, Vol 32, Issue 7, July 2020
[37] Scott, R., Hypersonix, Kratos, team to develop and fly DART AE hypersonic vehicle, Janes, Jan 2022
[39] Jia, Y., et. al., Additive Manufacturing of ZrB2-ZrSi2 Ultra-High Temperature Ceramic Composites using an Electron Beam Melting Process, Ceramics International, Volume 47, Issue 2, Jan 2021
[40] Feilden, E., et. al., High Temperature Strength of an Ultra-High Temperature Ceramic Produced by Additive Manufacturing, Ceramics International, Volume 45, Issue 15, October 2019
[41] Ionescu, E., et. al., Polymer-Derived Ultra-High Temperature Ceramics (UHTCs) and Related Materials”, Advanced Engineering Materials, June 2019
[42] Ciu, H., Hensleigh, R., et. al., Additive Manufacturing and Size-Dependent Mechanical Properties of 3-D Microarchitected High-Temperature Ceramic Materials, Journal of Materials Research, Feb 2018
[43] Duffy, R., Q&A with Radian CEO Richard Humphrey, Payload Space, Jan 2022
[44] Jones, A., Chinese Space Plane company targets suborbital tourism, point-to-point travel by 2025, Space.com, Jan 2022
[46] www.sbir.gov/sbirsearch/detail/1936637
[48] https://aviationweek.com/aerospace/spike-takes-different-path-supersonic-renaissance
[50] Mullins, C. and Lehmer, R., Executive Summary of ‘Independent Market Study: Commercial Hypersonic Transportation’, BryceTech and SAIC, Feb 2021, NTRS Document ID 20210015472
[51] Mullins, C. and Lehmer, R., Independent Market Study: Commercial Hypersonic Transportation, BryceTech and SAIC, April 2021, NTRS Document ID 20210015471
[52] Bastedo, W., et. al., T036 Hypersonic Study: Industry Presentation, Deloitte and SpaceWorks and the National Institute of Aerospace, Feb 2021, NTRS Document ID 20210014932
[53] Bastedo, W., et. al., Commercial Hypersonic Transportation Market Study, Deloitte and SpaceWorks and the National Institute of Aerospace, April 2021, NTRS Document ID 20210014711
[54] https://dod.defense.gov/Portals/1/Documents/pubs/2018-National-Defense-Strategy-Summary.pdf
[55] https://crsreports.congress.gov/product/pdf/R/R45811
[56] https://breakingdefense.com/2021/02/pentagon-hypersonics-director-rebuts-the-critics-point-by-point