Water Treatment
Erin Picton, PhD, Fellow
March 2024
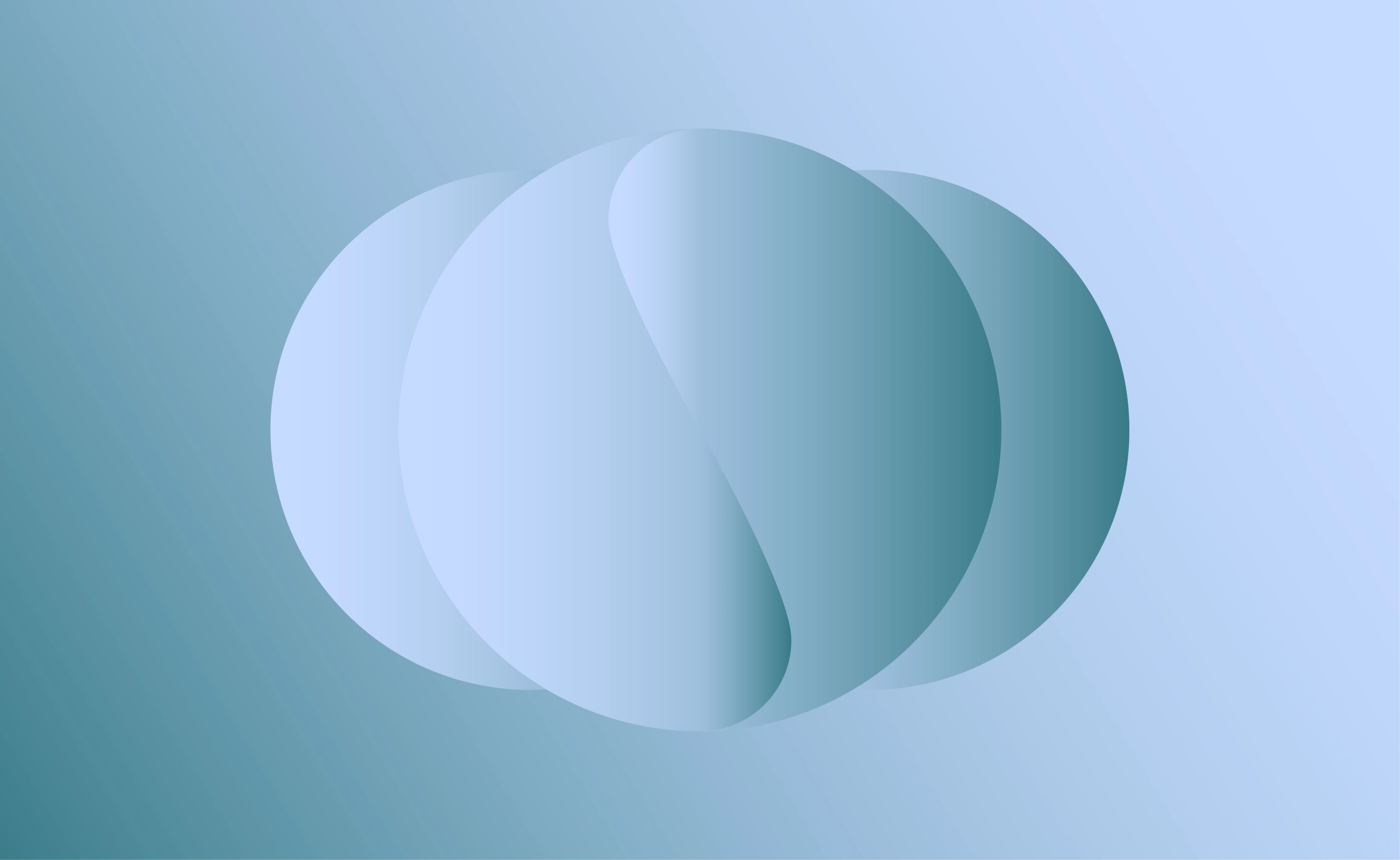
Introduction
Is the world running out of water? Ask a farmer in west Kansas praying for rain as their irrigation well dries up and the answer may be very different than a kayaker in Minneapolis gliding down the northern Mississippi River. But the reality is that we are facing a global water crisis in which the US is no exception. Just like carbon emissions produced in Europe affect the climate of someone living in Brazil, the ripple effects of water pollution and overconsumption reach further than our own backyards, especially with increasingly common drought events due to climate change. Shifting cultural sentiment around water is slow but signals of change are cropping up with increasing frequency. In the investment community, entire venture funds like Burnt Island and PureTerra are built around water industry investments. From a policy standpoint, the Infrastructure Law in the US invested more than $50 billion in water, and UN sustainable development goal 6 directs policymakers to ensure water access by 2030.[1, 2]
Popular culture is increasingly bringing water conversations to the water cooler and the dinner table. Sci-fi flicks like Mad Max: Fury Road (2015) and Dune (2021) paint vivid pictures of dystopian worlds without water. Documentary films like Blue Gold (2008), Brave Blue World (2020) among others, highlight water crises and some of the industries doing more than their share to accelerate them. Recall the ominous outro of the box office hit The Big Short from 2015 regarding Michael Burry: “The small investing he still does is all focused on one commodity: water.” Michael, played by Christian Bale, famously predicted the subprime mortgage crisis years before others. What had Michael betting on water in the last decade and where might it be worth doubling down?
Background
Conventionally, water that makes its way to the tap originates from one of two sources, surface water or groundwater, with saltwater from the ocean coming in a distant third place via desalination.
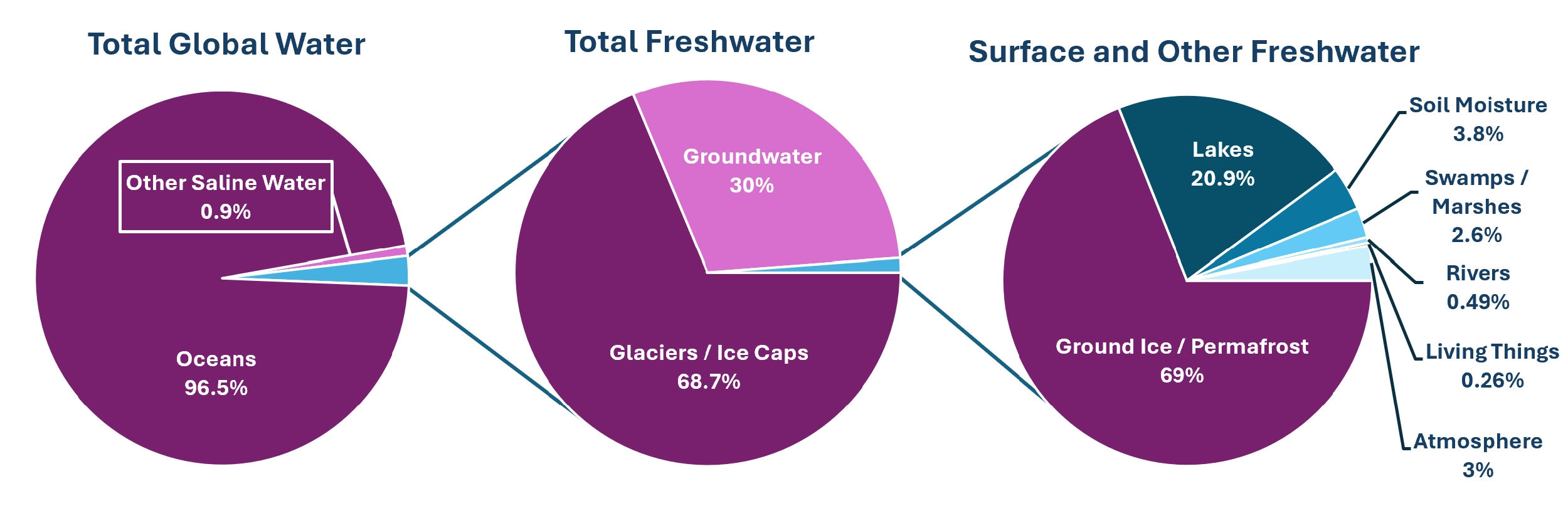
Figure 1: Global distribution of water resources Source: USGS [3]
Surface freshwater accounts for about 80 percent of the water consumed by humans and flows through lakes, rivers and streams at the earth’s surface.[4]
Groundwater is stored beneath the surface of the earth in aquifers and brought to the surface using wells. Historically, groundwater was seen as an almost limitless source but in fact groundwater is the most over extracted natural resource, as scientist Peter Gleick poignantly notes in The Three Ages of Water.[5] Some aquifers can be recharged as rainfall filters through the ground but in many places, urban development has decreased the permeability of the surface, causing water to run off instead of infiltrating down through the soil. As these aquifers are drained, formerly reliable water supplies run dry.
Ocean saltwater, the dominant form of water on earth, accounts for about 1% of drinking water.[6] This steadily climbing, yet modest slice of the pie has been enabled primarily by technological advances in Reverse Osmosis membranes over the past 3 decades. Desalination technology remains energy and capital cost intensive but membrane technology continues to improve, steadily driving down energy demand, installation costs, and replacement costs.
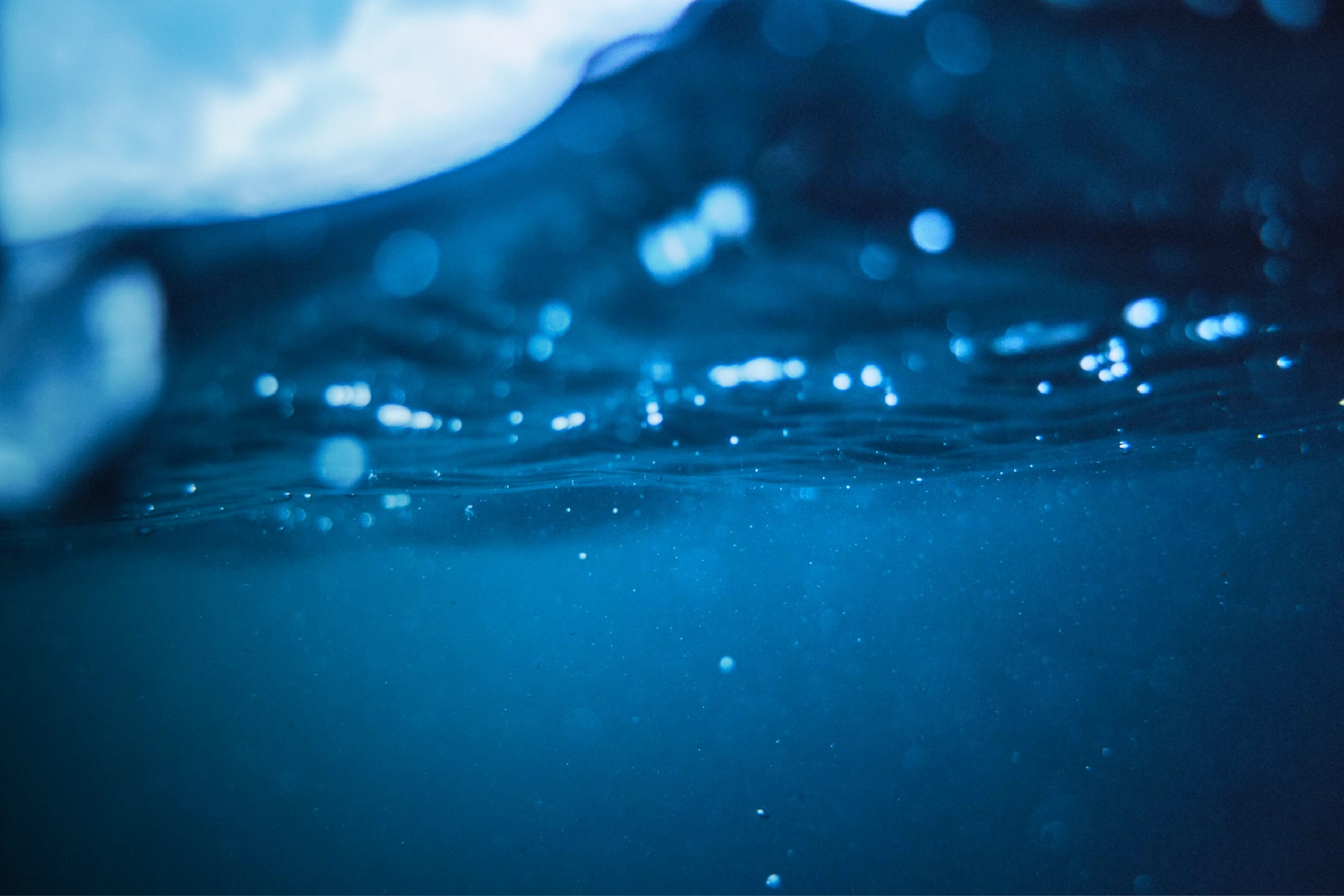
Conventional Systems
Conventional drinking water treatment infrastructure was designed and constructed at the beginning of the 20th century with a primary objective of eliminating acute infectious diseases caused by waterborne pathogens. These “Victorian era” processes, such as chemical coagulation, flocculation, and granular media filtration, have persisted to the current time because of their reliability and cost effectiveness. In the US, The Clean Water Act of 1972 accelerated the investment in wastewater treatment infrastructure in response to public concern about the declining quality of our nation’s lakes, rivers, and streams.
Water treatment upstream and downstream of the tap is decoupled and processed at two different facilities. Environmental water from surface water and groundwater supplies is treated at drinking water treatment facilities and distributed to consumers. The wastewater resulting from potable water use in our homes and businesses is collected in the sewer system and transported to wastewater treatment facilities for treatment, before being discharged to the environment.
The primary functions of drinking water treatment systems are filtration and disinfection, often with several unit operations upstream of these to aid in the effectiveness of these processes. In desalination plants, capital investment in RO membranes is so high that pretreatment will often resemble some version of a full conventional drinking water treatment system. The figure below is an example schematic of a conventional drinking water treatment system, a treatment process that is widely used, including at the City of Houston’s water purification plants. The water produced here is pumped throughout the community for household and commercial use.
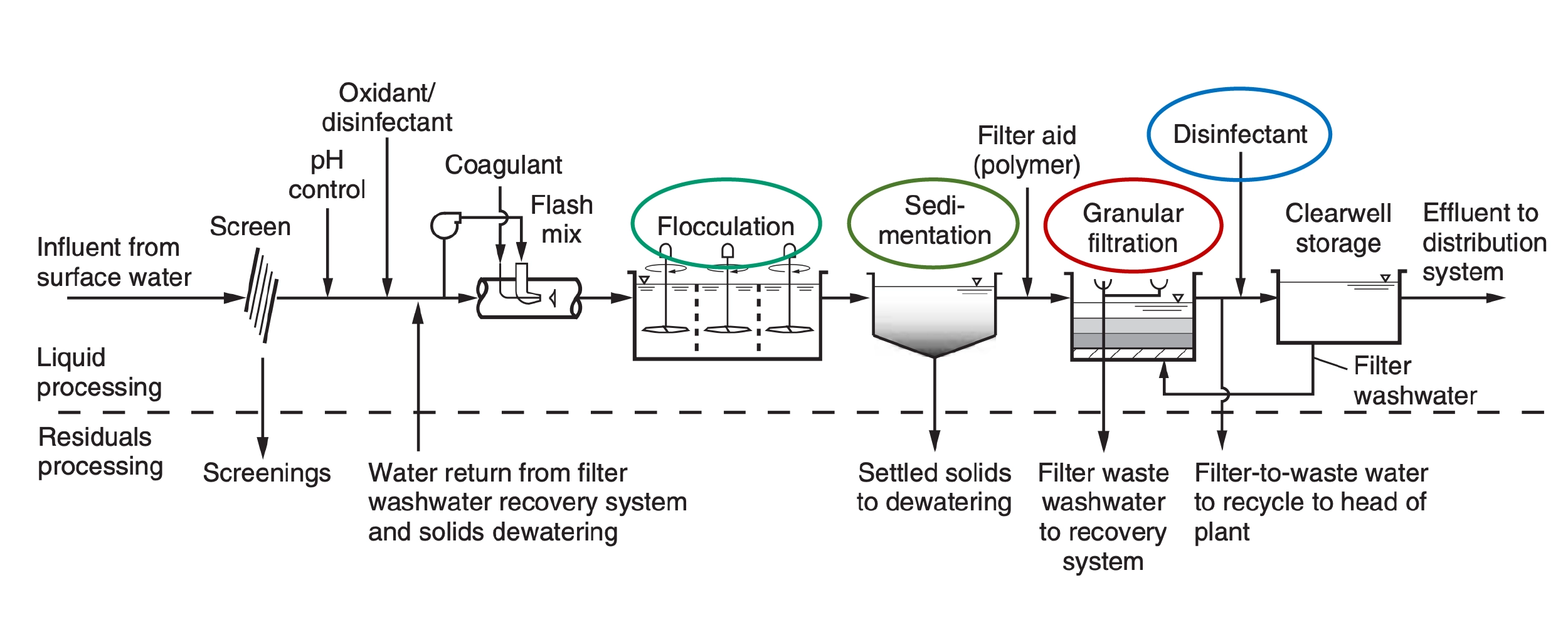
Figure 2: Source MWH's Water Treatment: Principles and Design, Third Edition: Principles and Design
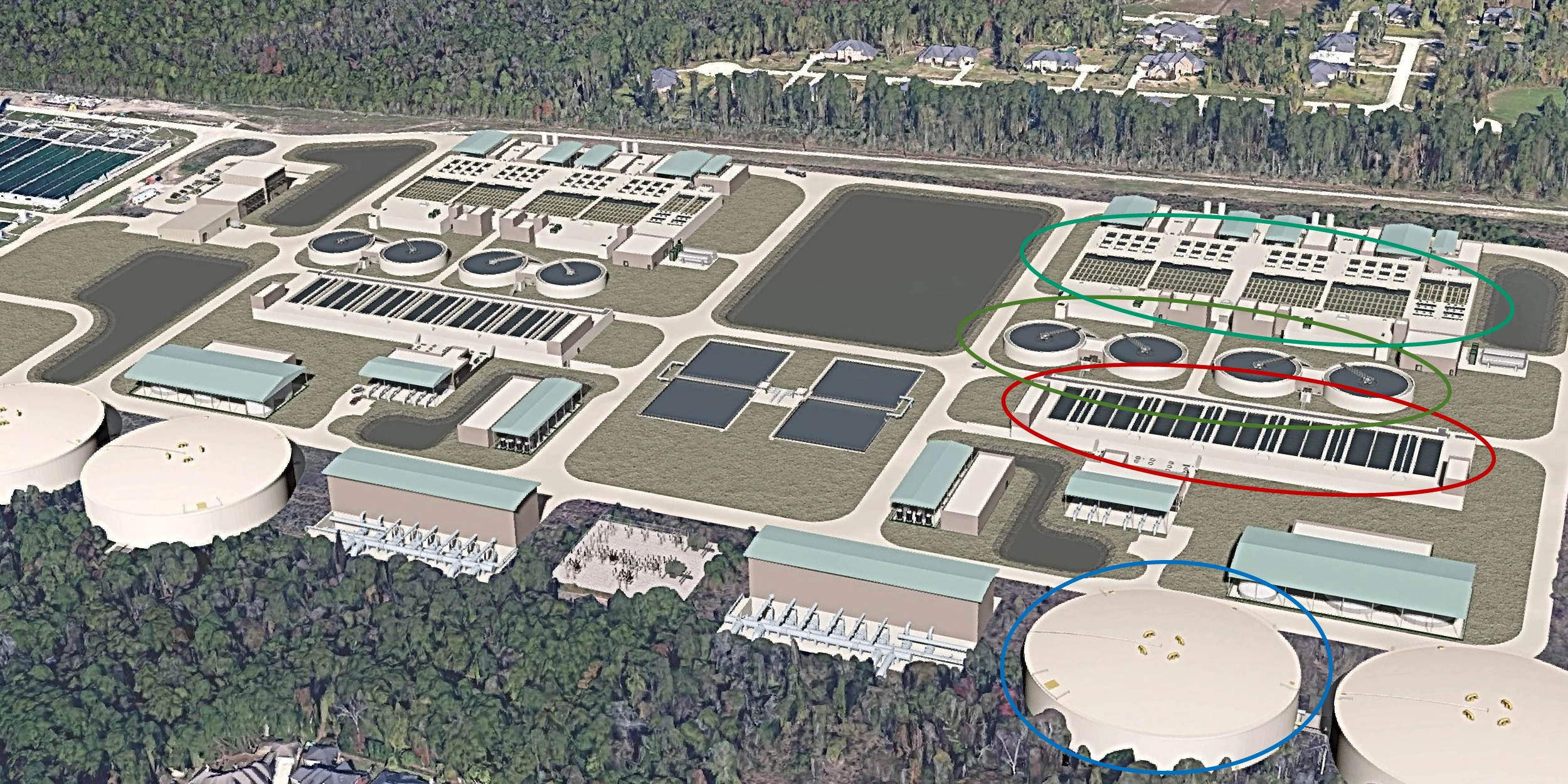
Figure 3: City of Houston; Northeast Water Purification Plant expansion, project rendering
From the distribution system the water arrives at the tap, and after use, goes down the drain and through the sewer system to a separate facility, the Wastewater Treatment Plant. WWTPs conventionally consist of sedimentation basins, some form of bioreactor, and disinfection. They produce treated water which, in most cases, is discharged to the environment and biosolids as a sludge byproduct which is destined for landfills or can be reused commercially as compost, for example.
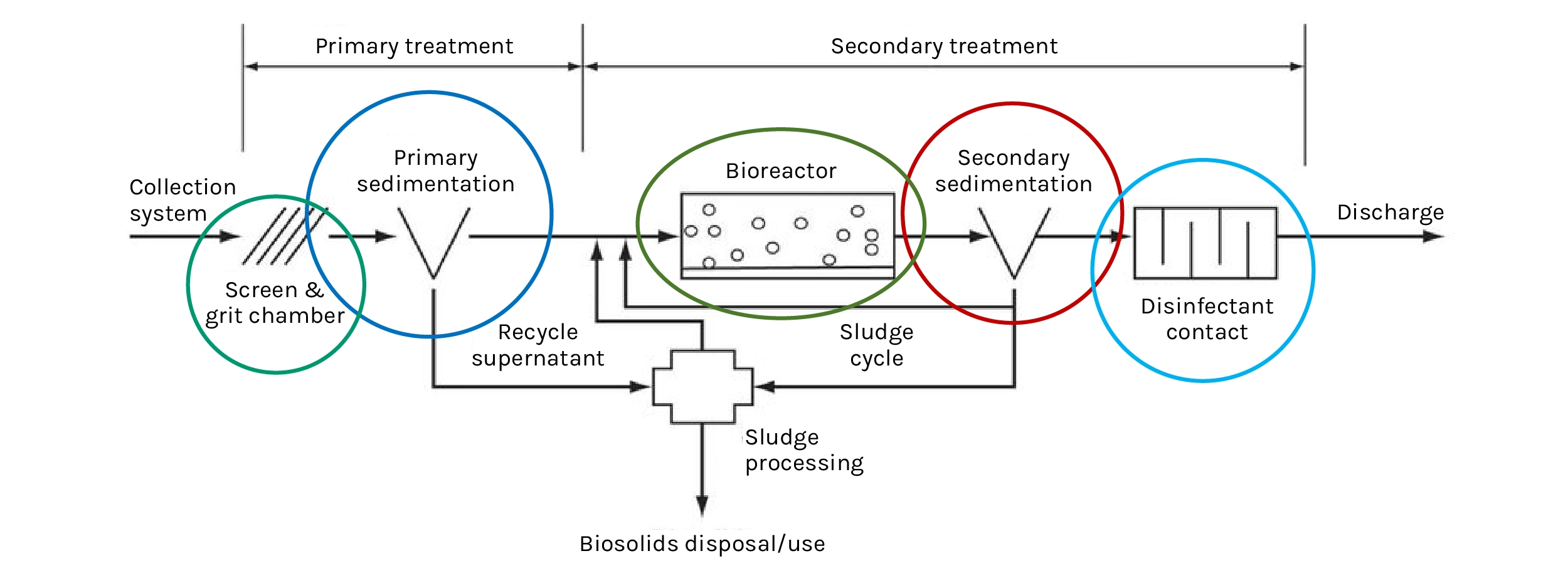
Figure 4: Introduction to Environmental Engineering and Science: Third Edition, Gilbert M. Masters and Wendell P., Ela 0-13-601837-8[7]
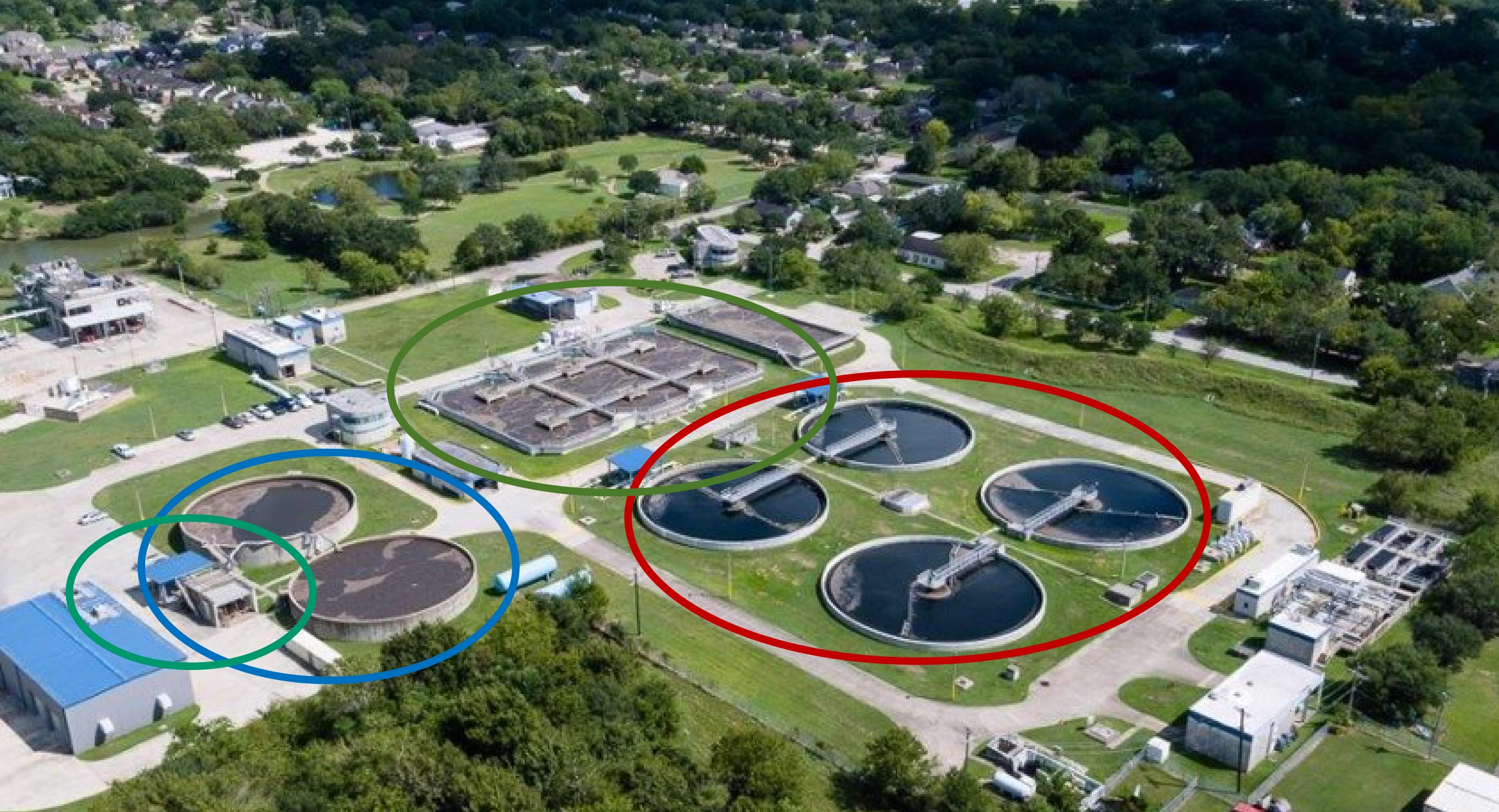
Figure 5: The city of League City, TX[8]
Challenges
Several problems exist with the status quo, many of which demand policy changes, societal shifts, and industrial oversight to protect human health, the environment and a sustainable economy. Through conversations with industry experts and startup founders three major challenges stand out, as well as three major trends in technology which may help address these issues for a sustainable water economy in the future.
Challenge 1: Emerging Contaminants
Emerging Contaminants are human-made and were not part of environmental water systems in the middle of the last century when most of today's drinking water treatment infrastructure took shape, therefore requiring modern technological solutions.
PFAS per- and polyfluoroalkyl substances are a class of chemical compounds found in household and industrial products as well as firefighting foams which are heavily used in aviation facilities. PFAS are heralded as “forever chemicals” which can be harmful to human health even at very low levels of exposure (parts per trillion).[9] On April 10, 2024, the US EPA set maximum contaminant levels (MCL) for PFOA and PFOS at 4.0 parts per trillion (ppt). This addition to the national drinking water standard makes PFAS/PFOA limits legally enforceable at the federal level and has been on the roadmap since 2021, driving innovation and investment remediation technologies.[10]
PFAS remediation technology can be broken into 3 steps, detection, concentration and destruction. Testing for PFAS is challenging because it is measured in nanograms per liter (parts per trillion, or ppt) which requires large, expensive scientific equipment and founders have reported lead times from third party laboratories of up to 6 months. There is a significant opportunity in real time field testing and accelerated lab testing that can help stakeholders make informed decisions about PFAS. Startups are emerging and pivoting to meet this opportunity. For example, Fred Sense have adapted their electrochemical field sensing hardware to meet this opportunity.
Once PFAS has been detected, it needs to be removed and disposed of or destroyed. It is advantageous for PFAS to be removed at high concentrations to keep subsequent disposal or destruction process liquid volumes low. The concentration step has an existing commercial solution and is typically done using methods like foam fractionation and air floatation. From here, the concentrated PFAS residual is disposed of as hazardous waste or fed to a PFAS destruction unit.
When evaluating PFAS destruction technologies, it is important to ask the question “which PFAS are being destroyed” because there are many different types of PFAS and the molecules can vary significantly in size and shape.
Some technologies rely on these molecules coming into contact with a reactive surface or an air bubble. Thus, they are often mass transfer limited and may struggle with capturing and destroying short chain PFAS. Smaller chains are less hydrophobic and prefer to stay in the water. And, there are fewer chances that the molecule will touch the active surface, allowing the reaction to occur. This is one reason companies like Aquagga destroy the PFAS in a bulk hydrothermal alkaline process. Other technologies like Axine Water Technologies use innovative reactor configurations in their electrochemical oxidation process to tackle this mass transfer challenge.
Microplastics are now ubiquitous in the environment and come from a variety of sources including the breakdown of larger plastics over time. Conventional drinking water treatment systems and filtration units have proven effective in removing particles in the same size range as microplastics, however there are other pathways for microplastics to enter the body. Intuitively, humans could ingest microplastics via water in contact with plastic water bottles. Less intuitively, microplastics could be in the food we eat as environmental microplastic contamination makes its way from bodies of water to fish, plants and livestock which are consumed by humans.
Importantly, removing microplastics from wastewater before discharge to the environment becomes critical. In the biological processes used for domestic wastewater treatment, the vast majority of microplastics are removed in the process biosolids. On the other hand, in a landmark ruling, a 2019 Texas court found that Formosa Plastics was in violation of the Clean Water Act for discharge of plastic pellets into waterways and issued a stringent consent decree demanding “zero” plastic discharge from its refinery on the Texas coast. The company is still working to meet these new requirements, coming up against technological limitations. If this is the new standard for plastics in industrial wastewater, plastic manufacturers will be motivated to implement new technologies to avoid fines to the tune of millions of dollars per month.
Active Pharmaceutical Ingredients are another growing source of industrial pollutants with yet untold magnitudes of environmental impact. Discharge from the wastewater of pharmaceutical manufacturers is regulated and disposed of as hazardous waste, often via incineration which produces emissions and carries a staggering price tag. While this problem may seem straightforward to head off at the source through monitoring, regulation, and treatment of industrial discharge, this is not the only source of APIs. They are increasingly concentrated in landfills and in municipal wastewater due to consumer disposal or excretions of still-active drugs in urine. Effects on the environment and human health can include disruption to the health and behavior of animal species, including the well reported study of changes to the sexual organs of frogs from a commonly identified hormone in wastewater. Physicochemical and microbial approaches to destroying APIs in wastewater are active research areas. Axine Water Technologies is able to divert APIs from incineration, avoiding significant costs and carbon emissions in the process.
Notable PFAS Companies
Aquagga Hydrothermal Alkaline Treatment for Complete PFAS Destruction
Axine Electrochemical oxidation for PFAS destruction
Aclarity Electrochemical PFAS destruction
Revive Environmental Supercritical water oxidation to destroy PFAS
Fred Sense Portable PFAS detection field kits
Allonia PFAS sensor test kit and PFAS removal via foam fractionation
Challenge 2: Increased Physical Scarcity coupled with Increased Demand
Supply
Groundwater aquifers are being depleted at alarming rates. Climate change is causing increased atmospheric temperatures and increased frequency and intensity of severe weather events, sustained droughts included. In India, where agricultural practices rely heavily on groundwater, warming temperatures are increasing withdrawals which researchers say could continue to accelerate.[11]
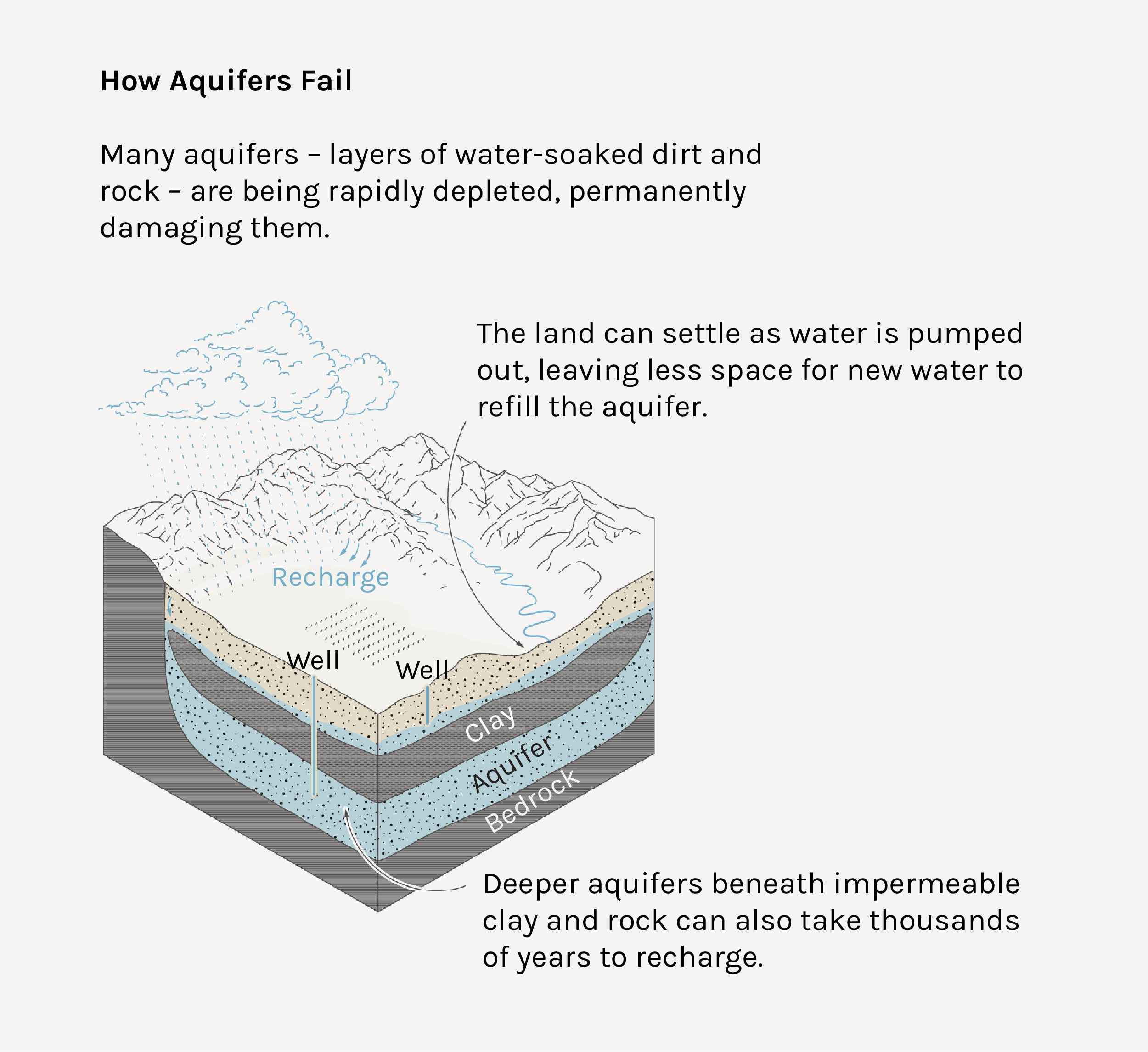
Figure 6: How Aquifers Fail. Source: New York Times
When the rate of extraction exceeds the rate of recharge, groundwater “mining” occurs. In some geologic settings, the cities perched atop these aquifers to sink into the ground as the depleted aquifer compresses. This phenomenon is called “subsidence” and in extreme cases like Mexico City can occur at rates of nearly 2 feet per year. Subsidence aside, there are supply limitations to meeting water demand through groundwater mining. For example, in Arizona, growing communities must demonstrate an assured 100-year water supply, which may not be possible through groundwater mining alone. By necessity, Arizona is very advanced in its water supply monitoring and regulations.
Surface water supplies are also facing challenges. The Panama Canal relies on fresh surface water supplies for its operation and drought in early 2024 has caused severe disruptions to one of the world’s most important trade routes.[12] Here in the US, water scarcity in the Mississippi River watershed has been historically high, triggering the White House to issue an emergency declaration for New Orleans and surrounding areas in September 2023 as salt water intrusion from the gulf of Mexico threatened drinking water supplies.[13] An official “shortage” was declared in the Lower Colorado River Basin for the first time in August 2021, triggering reduced allocation to Arizona and Nevada. Underground storage of surface water supplies, shifting allocations from agriculture to domestic and industrial uses, and groundwater withdrawals can temporarily meet demand, but more sustainable long-term management strategies must be identified.
Future demands will be met through supply-side and demand-side management.
Demand
In November 2022 the population of earth reached 8.0 billion people, up from 2.5 billion in 2050,[14] and growth projections estimate a population of around 9.7 billion by 2050. A surging global population and modernization, including changing dietary preferences are expected to increase future water stress.[15]
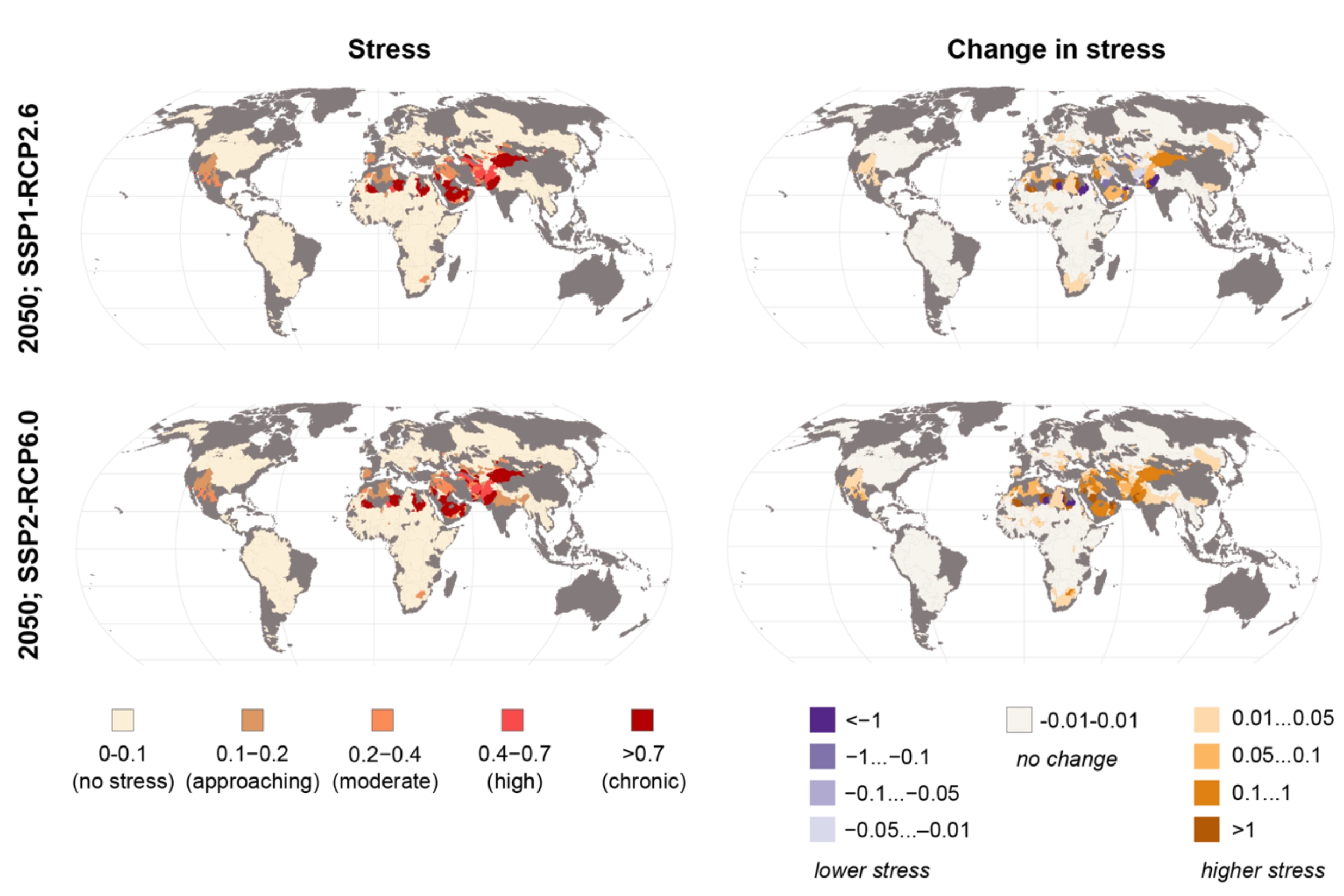
Figure 7: Current water scarcity and the projected change in water stress by 2050 in two different scenarios. The top scenario assumes climate change is held to 2°C and low population growth, while the bottom depicts high rates of population growth and a slow carbon emissions response. Source[16]
In some cases, technological advancements may accelerate the need for demand management. For example, the rise of AI has caused enormous spikes in water usage by Microsoft and other major tech companies as they race to develop generative AI and large language learning models like chat GPT.[17] Data centers use water for cooling and in these cooling systems, energy and water efficiency are often at odds with each other, the cooling systems are becoming more intelligent but innovation is needed to help companies like Meta and Microsoft meet the pledge of water positivity by 2030.[18, 19, 20] Another example lies in the demand for cooling water associated with green hydrogen production which is higher than low emission pathways, highlighting the frequent tradeoff between energy consumption/water consumption or put another way,
carbon footprint vs water footprint.
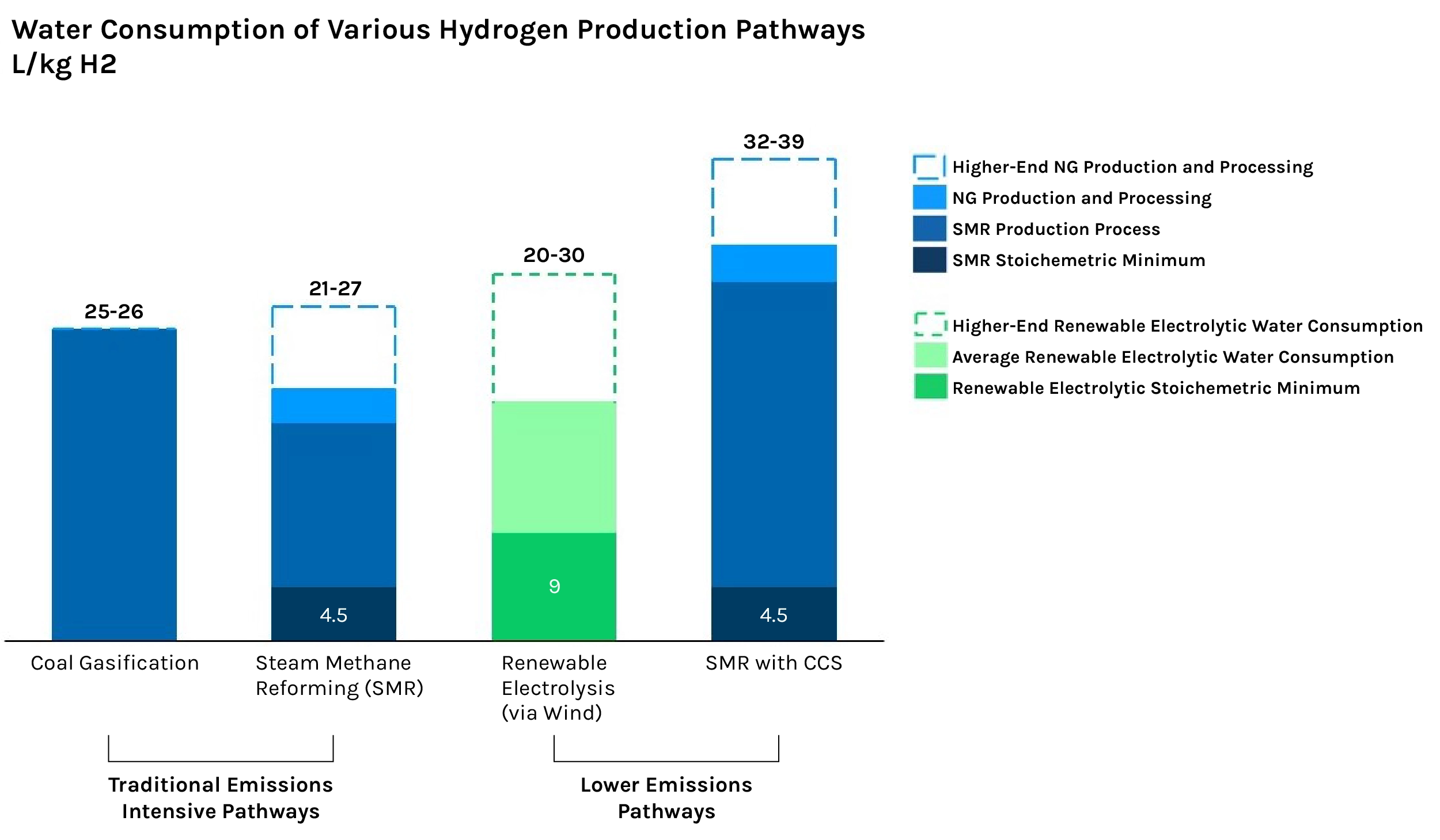
Figure 8: Rocky Mountain Institute: Reducing carbon footprint by producing low emission hydrogen may increase water footprint compared to traditional pathways. Source[21]
Notable Desalination Startup Companies
Wacomet Switchable solvent desalination
Membrion Electro-ceramic desalination for harsh industrial wastewater
Zwitterco Membranes designed for high – fouling environments
Eden Tech Reverse Osmosis Centrifuge (RCO)
Nona+Technologies Electro membrane Ion Concentration Polarization (ICP)
Challenge 3: Wastewater Disposal Costs
The US Clean Water Act was passed in 1972 to help protect the nation’s waterways. Since then, industry has been under increasing pressure to responsibly dispose of its wastewater, which amounts to significant operational expense. The degree to which the provisions of the clean water act are enforced varies regionally. In extreme cases cited by startup founders, wastewater is removed from an industrial process via tanker truck and travels hundreds of miles to an incineration facility. The operational costs of these types of operations are staggering, let alone carbon footprint! Disposal operations vary in operational intensity but generally tend to be a motivating factor for investing in new water treatment technology.
Notable Wastewater Startup Companies
Epic Cleantec Building wastewater recycling
EnBiorganic Scalable microbe growth in onsite bioreactors
Trends
Trend 1: Circularity and Wastewater Valorization
As climate change and population growth usher in a new age of water scarcity, the linear systems of the past are increasingly being replaced by circular economies. This is a trend we have seen across the industrialized world, partially driven by concerns for human health and the environment in the case of plastics and partially by economic factors like scarcity of raw materials in the case of lithium ion batteries, for example. Wastewater circularity is not a new concept, in fact Namibia was doing this several decades ago with sophisticated municipal wastewater to drinking water treatment systems developed in the 1990s. Since then Spain, the US, Israel, Singapore and others have joined the ranks of water stressed regions implementing circular water systems. An idealistic future features circular systems to reduce water consumption across the board, but
practical applications tend to begin in fit-for-purpose industrial sectors due to high costs of wastewater disposal and associated desire to keep disposal volumes low.
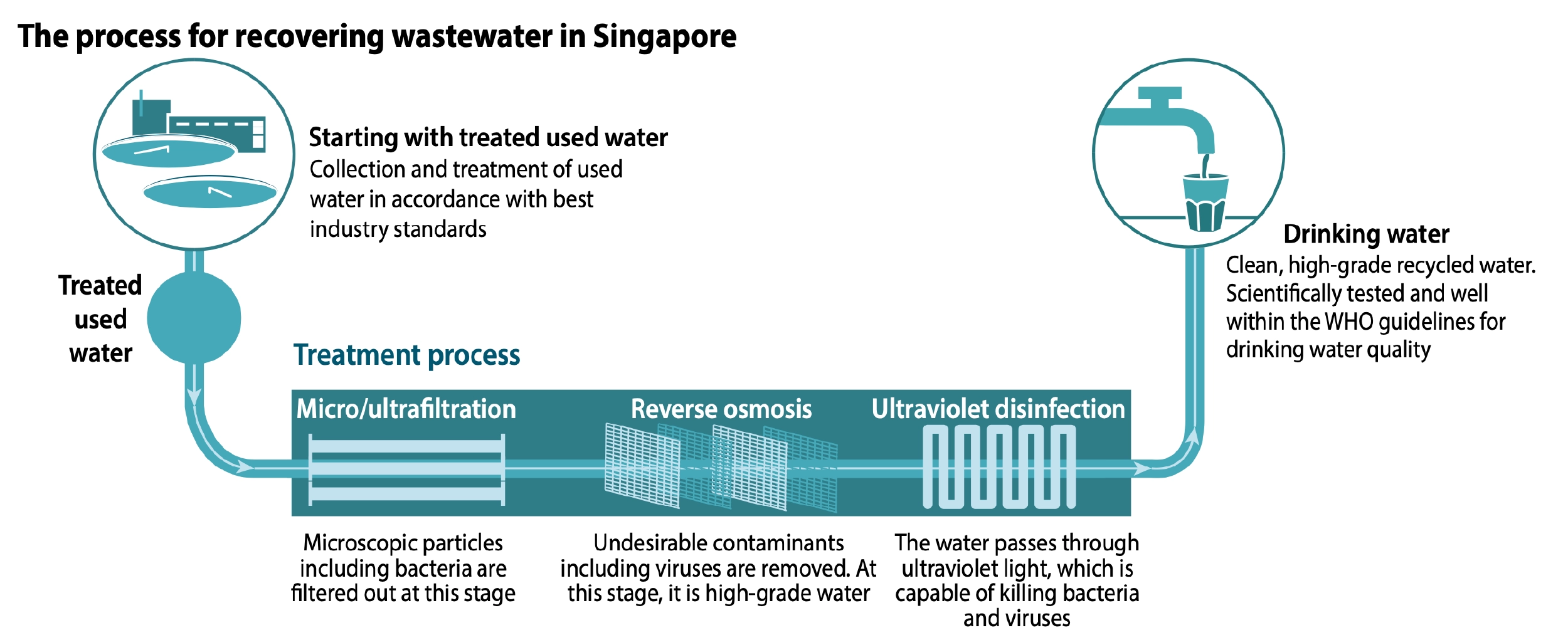
Figure 9: The Process for recovering wastewater in Singapore. Source: UN Environmental Program[22]
Building on the circular water systems of the past, innovators are now looking to recover not only the water, but also potentially valuable resources in the water including rare earths, lithium, copper, and organic compounds which can be used in agricultural or pharmaceutical applications.
Water treatment technologies tend to produce two streams from the treatment process, a clean, treated water stream and a high concentration brine or sludge stream. For example, about 50% of the salty ocean water that enters a reverse osmosis plant comes out as fresh, drinkable water but the other 50% is rejected into a hypersaline brine waste product. Even when the RO plant is next to the coast, the discharge of this concentrated brine back into the ocean presents ecological problems, and these challenges are compounded exponentially for inland facilities. Several startup companies are working on ways to treat this waste stream to produce more fresh water and eliminate the problem of contaminated liquid discharge. RO is not the only industry that produces too-salty wastewater, the oil and gas industry for example has enormous infrastructure and disposal wells to manage produced water, a hyper saline brine which flows to the surface along with oil and gas. Treatment technologies in these markets are competing with the cost of disposal which often includes drilling a disposal well and injecting the waste product underground.
Key Questions
- What is the levelized cost of water (LCOW[23]) at scale and how does this compare to the current disposal costs + current water purchase costs?
- If valorization of nutrients or minerals is being considered, what is the cost to produce these conventionally, and how does the valorization process compare?
Trend 2: Decentralization or “Water on the Edge”
68% of the population is expected to live in urban areas by 2050. The rush of humanity into cities presents problems for conventionally designed water treatment infrastructure, if the population growth exceeds the design limits, retrofitting treatment, storage basins and distribution pipelines to keep up with this growth is not necessarily feasible. This presents an opportunity for modular, self-contained water treatment systems to handle “edge” cases and relieve the central plant of demand spikes. Similar decentralized technology could be translatable to remote, off-grid applications.
In developing countries which may not have been designed around the conventional water treatment systems we are accustomed to in western cultures, there is an opportunity for leapfrogging.[24] For example, many African countries bypassed land-line phone infrastructure and went straight to cell phones.[25] Companies like Off Grid Box employ a sustainable energy and water kiosk model for off grid operations in remote areas. Look out for other opportunities for investment in these markets where willingness to adapt and public needs are high.
Key Questions
- What is the LCOW? The department of energy set LCOW targets for decentralized, small plant desalination at $1.50/m3.
- How does the maintenance and durability of the system lend itself to decentralized and off-grid operations?
- What regulatory environment can encourage private sector intervention while also maintaining consumer confidence in private water suppliers?
Trend 3: Innovative Water Valuation Structures
If it is cheaper to pull water out of a nearby river than to recover it from waste, businesses and cities will choose the former. New technology for a sustainable water economy is attractive, but when everything comes down to cost the value proposition falls apart. But who is responsible when, hundreds of miles away in the same watershed, the river dries up and there is not enough flow to supply drinking water to a city? What are some ways to get more businesses thinking about the bigger picture of water scarcity?
One possible path is through the emerging interest in tokenized water. Water markets like waterDAO and others are being developed to drive water innovation. Similar to voluntary carbon credits, consumers concerned about their water footprint can purchase a “water credit” to offset their water use with verified water production or new technology development. Like with carbon credits, blockchain technology could similarly be used to bring greater transparency and trust to these transactions. Carbon offsets and the voluntary carbon market have driven substantial advancement of nature based and technological carbon capture and sequestration. It is worth noting that, unlike carbon in the atmosphere, water resources and water stress are more localized, so spatial constraints would be critical in ensuring the effectiveness of such systems. Still, this type of supplemental market force could help drive down the LCOW for emerging technologies and offer a solution to water consumers for whom it is operationally impossible to reach net zero water.
Key Questions
- How will permanence, additionality, chain of custody, and regionality be verified?
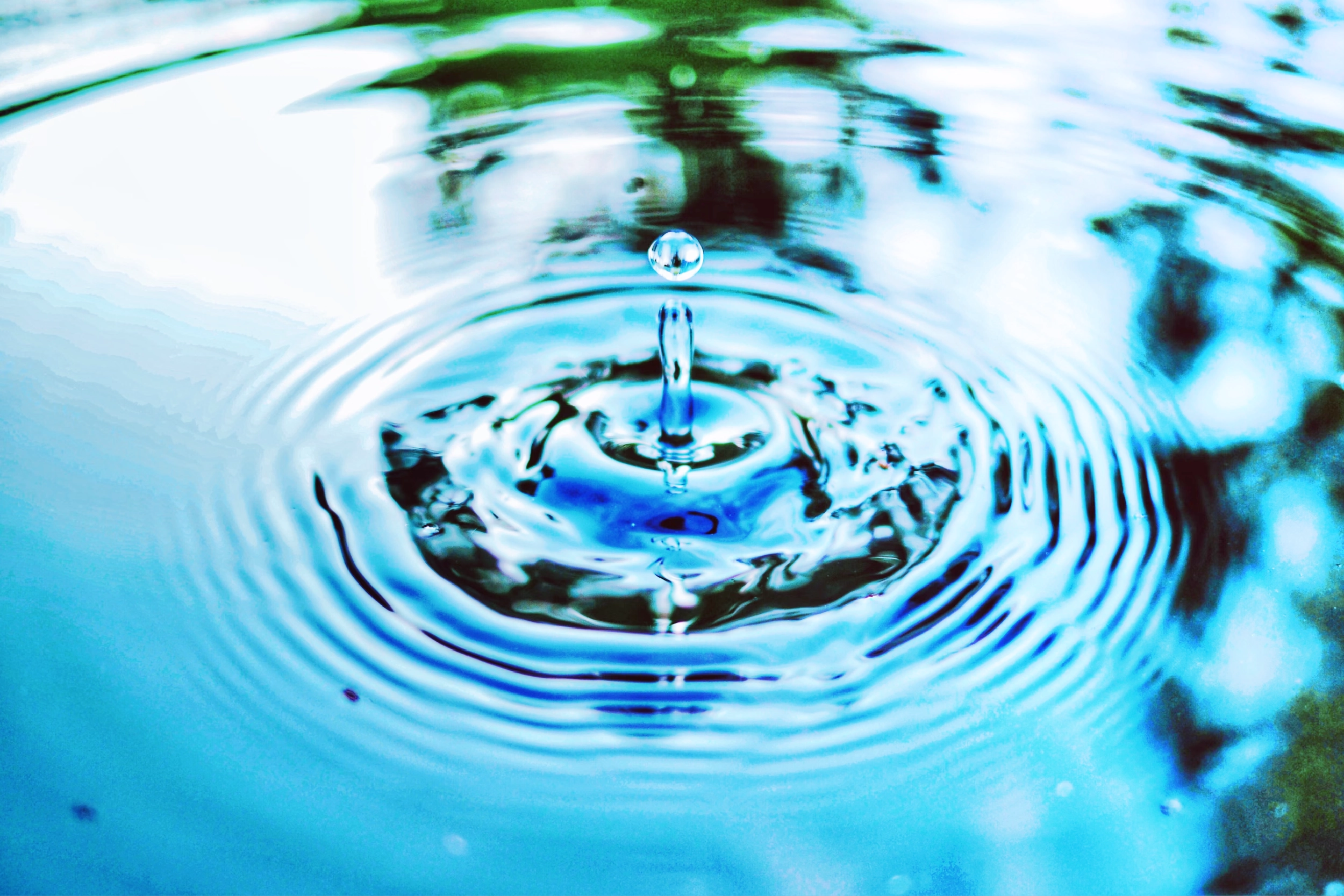
Insights
- Technologies that can reduce freshwater withdrawals by harvesting water from waste should seek to minimize liquid discharge, ideally targeting zero. Solids residuals from these processes are more easily managed, and potential negative environmental impacts are more localized.
- Keep the levelized cost of water in mind – what is the company strategy to compete with a low-cost water market? Wastewater disposal costs can be very motivating!
- Consider the long-term stability of the regulatory environment to sustain willingness and ability to pay for new technology.
- Reliable testing and monitoring for emerging contaminants is a bottleneck with plenty of room for innovation.
Notes
[1] Bipartisan Infrastructure Law: A Historic Investment in Water, US EPA
[2] Goal 6: Ensure access to water and sanitation for all, United Nations
[3] Where is Earth's Water?, USGS
[4] Information on Earth’s Water, NGWA
[5] The three ages of water: Prehistoric past, imperiled present, and a hope for the future (Gleick, 2023)
[6] Desalination – Past, Present and Future, International Water Association
[7] Introduction to Environmental Engineering and Science: Third Edition Gilbert M. Masters and Wendell P. Ela 0-13-601837-8
[8] The city of League City, TX
[9] Our Current Understanding of the Human Health and Environmental Risks of PFAS, US EPA
[11] Nishan Bhattarai et al. ,Warming temperatures exacerbate groundwater depletion rates in India.Sci. Adv.9,eadi1401(2023).DOI:10.1126/sciadv.adi1401
[12] Panama Canal traffic cut by more than a third because of drought, AP World News
[13] President Joseph R. Biden, Jr. Approves Louisiana Emergency Declaration, The White House
[14] Global Issues: Population, United Nations
[15] Tompa O, Lakner Z, Oláh J, Popp J, Kiss A. Is the Sustainable Choice a Healthy Choice?-Water Footprint Consequence of Changing Dietary Patterns. Nutrients. 2020 Aug 25;12(9):2578. doi: 10.3390/nu12092578. PMID: 32854386; PMCID: PMC7551173
[16] Munia, H. A., Guillaume, J. H. A., Wada, Y., Veldkamp, T., Virkki, V., & Kummu, M. (2020). Future transboundary water stress and its drivers under climate change: A global study. Earth's Future, 8, e2019EF001321. https://doi.org/10.1029/2019EF001321
[18] Making AI Less “Thirsty”: Uncovering and Addressing the Secret Water Footprint of AI Models
[19] Restoring More Water Than We Consume by 2030, Meta
[20] Microsoft will replenish more water than it consumes by 2030, Microsoft
[21] Hydrogen Reality Check: Distilling Green Hydrogen’s Water Consumption, Rocky Mountain Institute
[22] Wastewater - Turning Problem to Solution, UN Environment Programme
[23] Pipe Parity Analysis of Seawater Desalination in the United States: Exploring Costs, Energy, and Reliability via Case Studies and Scenarios of Emerging Technology. Hunter Quon, Joshua Sperling, Katie Coughline, David Greene, Ariel Miara, Sertac Akar, Michael Talmadge, Jennifer R. Stokes-Draut, Jordan Macknick, and Sunny Jiang, ACS ES&T Engineering 2022 2 (3), 434-445, DOI: 10.1021/acsestengg.1c00270
[24] Why Africa Has The Ability To Leapfrog The Rest Of The World With Innovation, Forbes
[25] Leapfrogging : the key to Africa's development - from constraints to investment opportunities, The World Bank